Physiology of the Preterm and Term Infant
Timothy M. Hoffman
Stephen E. Welty
Introduction
Approach to the care of the neonate with congenital heart disease is multidisciplinary. Regardless of where the patient is cared for (neonatal, pediatric, or cardiac intensive care unit) a firm understanding of neonatal physiology and development by the managing medical team is imperative to provide state-of-the-art interdisciplinary care. This chapter provides a unique perspective of the neonate from a multiorgan system approach. Cardiac lesion–specific data are outlined throughout the textbook; therefore this chapter does not include a discussion of these conditions. Instead, this chapter focuses on the complex interactions of multiple organ systems in neonates who also have congenital heart disease.
Transitional Circulation
With the onset of spontaneous respiration at the time of birth, the low-resistance placenta is removed from the circulation, thus increasing systemic vascular resistance. Expansion of the lungs elicits an immediate decrease in the pulmonary vascular resistance as a result of physical recruitment of pulmonary vasculature and vasodilation of the pulmonary arteriolar bed in response to elevated oxygen content. In turn, the shift of the systemic and pulmonary vascular resistances causes a reversal of flow of the ductus arteriosus from right to left to predominantly left to right. In theory, this change from fetal circulation causes an increase in pulmonary blood flow and a decrease in systemic venous return due to the lack of umbilical venous flow. Left atrial pressure increases and eventually exceeds the pressure in the right atrium leading to closure of the foramen ovale flap against the crista dividens, eliminating shunting at the atrial level. All of these alterations may be influenced by disease processes that affect the systemic and pulmonary vascular resistances, inhibiting the usual transition to adult circulation (1,2). Additionally, after the initial precipitous fall, pulmonary vascular resistance continues to fall gradually in the first 48 hours of life and takes several weeks to decrease to adult levels. In a normal neonate, the ductus generally closes functionally within several days of life.
Patent Ductus Arteriosus
Classic studies by Gittenberger-de Groot have described the sequence of events that occur in the infants that lead to functional and anatomic closure of the ductus arteriosus (3). The molecular events explaining closure and the predisposition of the ductus to remain patent in premature infants have been described (4,5,6,7,8). Ductal closure is dependent upon an initial ductal constriction which results from the development of hypoxia within the ductal media. Hypoxia mediates a series of molecular events that then lead to disruption of the internal elastic lamina and endothelial cell. Smooth muscle cells proliferate forming intimal mounds that impinge on the ductal lumen, ultimately leading to its anatomic closure. In premature infants, ductal constriction is essential for anatomical closure of the ductus arteriosus. But this alone may not be sufficient for ductal closure. Hypoxia in the muscular media may not occur and the postconstriction sequence fails to follow. Thus, premature infants may present with a symptomatic patent ductus arteriosus (PDA), especially those who are <30 weeks of gestation.
The incidence of PDA in premature infants is inversely related to gestational age. In a multicenter trial of indomethacin prophylaxis in premature infants weighing <1,000 g, the incidence of PDA in the placebo group was 50% (9). Premature infants with a PDA may present with classic findings of a left-to-right shunting lesion. In this case, infants typically have a systolic murmur, increased left precordial activity, sometimes with an associated thrill, bounding pulses, and a wide pulse pressure that also may be evident on umbilical arterial waveform tracings. Unfortunately, many infants with a physiologically significant PDA may not have these findings. Thus there should be a high index of suspicion for a PDA even without classic physical findings in a premature infant who has lung disease. Doppler echocardiography is the gold standard for diagnosing a PDA, and should be performed before treatment decisions are made.
Physiology of the PDA is secondary to overperfusion of the lung and possibly underperfusion of the systemic circulation. Infants’ lungs are fully recruited at rest so that any increase in flow from left-to-right shunts predictably increases fluid filtration in the lung (10). Increased fluid filtration will lead to pulmonary edema if it exceeds the ability of the lymphatics to remove fluid, and pulmonary edema is frequently associated with pulmonary overcirculation from a
PDA. The PDA may also steal from the systemic circulation. Animal studies have shown that even small shunts can result in underperfused systemic organs (10). Studies in extremely premature infants with a hemodynamically significant PDA observed that although total left ventricular output was increased, flow was decreased in the abdominal aorta, celiac, mesenteric, and renal arteries, while there were no differences in the anterior cerebral artery flow (11). While the changes in acute physiology are concerning, the effect of a PDA on the outcome of premature infants and the management of a PDA remains controversial (12,13).
PDA. The PDA may also steal from the systemic circulation. Animal studies have shown that even small shunts can result in underperfused systemic organs (10). Studies in extremely premature infants with a hemodynamically significant PDA observed that although total left ventricular output was increased, flow was decreased in the abdominal aorta, celiac, mesenteric, and renal arteries, while there were no differences in the anterior cerebral artery flow (11). While the changes in acute physiology are concerning, the effect of a PDA on the outcome of premature infants and the management of a PDA remains controversial (12,13).
The management of the PDA in premature infants has changed dramatically over the last decade. The uniform findings from animal studies are that a PDA is associated with underperfusion of the systemic circulation and overcirculation of the pulmonary bed with the development of pulmonary edema (10,11). If these findings can be extrapolated to premature infants who weigh <1,000 g and approaches to preventing or treating the PDA lacked significant toxicity, it would support taking aggressive steps to prevent the development of a significant PDA, or at the very least, for treatment of these infants with a clinically significant PDA. Furthermore, epidemiologic data indicate that premature infants who develop a clinically significant PDA have higher rates of mortality and morbidity than do comparably sized infants who do not develop a significant PDA (14,15).
Treatment approaches include surgical ligation, pharmacologic treatment with cyclooxygenase inhibitors, or supportive medical management with fluid restriction, cardiovascular support, and therapy with diuretics. In 1996, an analysis of strategies including prophylaxis, early treatment, and late treatment after overt symptoms indicated that the most reasonable approach to the PDA in premature infants is using indomethacin either prophylactically in high-risk populations or within the first few days of life when the PDA became evident prior to the development of overt symptoms (16). However, there have been marked improvements in perinatal care including an increased emphasis on the treatment of mothers who are at risk for delivering a premature infant with antenatal steroids (17,18). Likewise a more aggressive approach to noninvasive respiratory support strategies allows even premature infants born <1,000 g to be frequently not supported with pressors or mechanical ventilation in the first 24 hours of life. In this changing clinical context, aggressive treatment of the PDA in an infant with minimal lung disease and support has come into question. A contemporary meta-analysis has concluded that prophylactic treatment of premature infants with cyclooxygenase inhibitors, while reducing the need for surgical ligation of the ductus and lowering the incidence of intraventricular hemorrhage, elicited no differences in survival or neurodevelopmental outcomes (19). Thus, a reasonable interpretation of the present clinical evidence supports the conclusion that the beneficial effect in closing the ductus in a population of infants who would have developed a symptomatic PDA may be offset by the deleterious effects of treating some infants who would not have developed a PDA with a potent class of drugs with a significant frequency of side effects. Thus, treatment of a hemodynamically significant PDA appears to be the most prudent approach in extremely low birth weight infants. Another meta-analysis compared indomethacin to ibuprofen which demonstrated no difference in efficacy in patients treated with ibuprofen, yet yielded a significantly lower incidence of side effects (20). This meta-analysis may lead to the conclusion that, at present, ibuprofen is the best treatment option for those with a symptomatic PDA. However, a subsequent analysis combining data from American and European studies found that ibuprofen therapy was associated with a greater risk of the development of bronchopulmonary dysplasia (BPD) than indomethacin therapy (21). Thus, the optimal choice of cyclooxygenase inhibitors remains unanswered.
Treatment of a PDA has taken a risk-based approach. If a premature infant with echocardiographic evidence of a PDA in the first week of life requires mechanical ventilation for support of lung disease that cannot be attributed to respiratory distress syndrome (RDS), treatment is warranted and a course of ibuprofen is probably the preferred choice. Furthermore, independent of the amount of cardiopulmonary support required, if a premature infant has clinical evidence of a PDA, such as wide pulse pressures, an active precordium, or a loud murmur and an echocardiogram demonstrates a ductus with evidence of left atrial or left ventricular enlargement, treatment is also warranted.
The role of surgical ligation in infants has become even less clear than the pharmacologic approach to treatment of a PDA. There is no evidence that early aggressive treatment with PDA ligation improves clinical outcomes (22), and recent evidence suggests that late ligation of a significant ductus arteriosus is associated with concerning long-term neurodevelopmental outcomes (23). These data do not exclude the potential role for surgical ligation, but rather may highlight the point that extremely premature infants who have a PDA evident after the first week of life define a population of infants at extremely high risk for long-term morbidity. However, based on the concerns about surgery, many clinicians choose to treat infants failing a course of cyclooxygenase inhibitors with fluid restriction and diuretics and defer ligation for the most symptomatic infants.
In short, there is ongoing debate and a cyclical scientific approach to the treatment of a PDA even in the extremely low birth weight infants. To further complicate the decision tree, catheter-based interventions, even for patients whom are extremely premature, are considered in select patients. As we approach the potential for catheter-based therapies, factors determining success include patient weight, ductal size and anatomy, and the availability of suitable device hardware (24).
Lung Development
Lung development from the embryonic phase to the alveolar phase has been studied in humans and in many mammals (25,26,27,28). Furthermore, the molecular basis for lung development continues to be elucidated and a discussion of the mechanisms for lung development is beyond the scope of this chapter. However, since lung development proceeds through postnatal life for several years, understanding the effects on lung development of congenital heart disease, its treatment, and supportive medical care for lung disease is important. Overall lung development can be optimized in infants with respiratory disease and heart disease whether they are born prematurely or not.
Infants born as early as 23 weeks of gestation can survive. At 23 weeks of gestation, infants are still in the canalicular phase of lung development, which continues through 26 weeks of gestation. Despite the relatively immature lung architecture, including no identifiable alveoli and a thickened alveolar interstitium with a double capillary network, the lung can sustain enough air exchange function for the infant to survive. The saccular phase of lung development extends from 27 to 36 weeks of gestation and the alveolar phase starts at 37 weeks and proceeds through approximately 3 years of postnatal age. In infants born prematurely without heart disease, supportive care with mechanical ventilation and supplemental oxygen frequently injures the lung and predisposes the infant to the development of chronic BPD (29). Furthermore, perinatal inflammation of the lung is frequently observed in premature infants, which also can injure the lung profoundly (30). The injury caused by lung support and/or inflammation leads to an arrest of lung development, and the lung function abnormalities can persist for years (31,32). Thus in infants, especially premature infants, who have heart disease and require supportive care delivered to the respiratory system, therapies should be pursued that limit lung injury and thereby limit aberrations in lung development, similar to treatment strategies being explored in infants born prematurely who do not have heart disease.
The most important intervention to improve the outcomes of premature infants is the administration of antenatal steroids to mothers who are at risk of delivering a premature infant. The
landmark study by Liggins and Howie demonstrated a beneficial effect of antenatal steroids given to mothers who delivered infants at <34 weeks of gestation (33). These results have been verified by many other randomized, double-blinded, placebo control trials. While the initial studies focused on decreasing the incidence of respiratory distress, there was also evidence that antenatal corticosteroid (ACS) administration decreased mortality and other morbidities in premature infants (34,35,36). ACS administration enhanced lung and circulatory development. So much so that an NIH consensus statement stated strongly that ACS should be given to mothers with threatened premature delivery between 24 and 34 weeks of gestation (17). Details for ACS administration can be found in the NIH consensus statement. There is no present evidence that supports repeat ACS administration to those mothers who do not deliver within 1 week of steroid administration (18).
landmark study by Liggins and Howie demonstrated a beneficial effect of antenatal steroids given to mothers who delivered infants at <34 weeks of gestation (33). These results have been verified by many other randomized, double-blinded, placebo control trials. While the initial studies focused on decreasing the incidence of respiratory distress, there was also evidence that antenatal corticosteroid (ACS) administration decreased mortality and other morbidities in premature infants (34,35,36). ACS administration enhanced lung and circulatory development. So much so that an NIH consensus statement stated strongly that ACS should be given to mothers with threatened premature delivery between 24 and 34 weeks of gestation (17). Details for ACS administration can be found in the NIH consensus statement. There is no present evidence that supports repeat ACS administration to those mothers who do not deliver within 1 week of steroid administration (18).
Surfactant administration has been shown to improve outcomes in premature infants. Exogenous surfactant has been given in prophylactic and rescue modes. In the prophylaxis studies of premature infants who were at high risk of having RDS, surfactant administration was given within 15 to 20 minutes of birth. This led to lower mortality rates and less morbidity than was seen in infants who were given surfactant after the diagnosis of RDS was established (selective) (37). These differences are less relevant in more mature infants. Thus, infants born after 30 weeks of gestation can be assessed for development of RDS and if RDS is present, surfactant should be delivered in the rescue mode (38).
In the last decade, as the antenatal steroid administration to mothers has increased and the expertise of noninvasive respiratory support has been enhanced, many infants, even extremely premature infants, are delivered with minimal lung disease so that a trial of noninvasive respiratory support with nasal continuous positive airway pressure (CPAP) may be reasonable. In 2008, the COIN (CPAP on intubation at birth) trial was published in which 610 infants between 25 and 28 weeks were randomized to either CPAP or intubation with surfactant at birth (39). While there were some differences favoring the CPAP group at 28 days of life, the outcomes at 36 weeks postmenstrual age for morbidity and mortality were not different. Another study by the SUPPORT study group (surfactant positive pressure and pulse oximetry randomized trial) enrolled 1,310 infants between 24 and 27 weeks of gestation which randomized similarly to the COIN study. The study results showed no statistically significant differences in the composite outcome of death or BPD at 36 weeks postmenstrual age (40). The combination of these findings suggests that infants born at 24 weeks of gestation up to 28 weeks of gestation who were exposed to maternal ACS and are vigorous at birth may be supported with CPAP without prophylactic surfactant. However, be the infants’ oxygen requirement rises to >40%, administration of surfactant should then be considered.
Other therapeutic measures in premature infants that help to prevent development of BPD include: (a) monitoring to prevent hyperoxemia and minimize exposure to supplemental oxygen and mechanical ventilation, (b) fluid restriction in the first few days of life (41), and (c) the institution of aggressive and early parenteral and enteral nutrition. The combination of therapies just discussed is supported in premature infants without congenital heart disease. However, there are no reports regarding the impact of congenital heart disease on lung development in premature infants, or on postnatal lung development in term infants. Acute management of premature infants with congenital heart disease is thus extrapolated from what has been observed in premature infants without heart disease.
Pulmonary Hypertension
Abnormalities of smooth muscle development frequently influence acute cardiopulmonary physiology in newborn infants. Pulmonary hypertension is frequently present when there is abnormal vascular smooth muscle development. Pulmonary hypertension presenting in the newborn period is classified into three separate categories based on the underlying mechanisms for the development of the disorder. The three categories include underdevelopment, maldevelopment, and maladaptive forms. In this classification only underdevelopment and maldevelopment pulmonary hypertension are associated with abnormalities of smooth muscle development. In fetal development, airway branching and smooth muscle development occur in parallel in an environment where pulmonary vascular resistance is high and blood flow is low. In normal lung development, smooth muscle development around the vasculature extends to the level of the respiratory bronchiole. When the fetus is compromised by poor placental function and high placental vascular resistance, smooth muscle development is altered so that it extends further distally in the pulmonary vasculature and is thicker. The thickening occurs as a combination of intimal and adventitial thickening which gives rise to maldevelopment pulmonary hypertension (42). Underdevelopment pulmonary hypertension is associated with pulmonary hypoplasia which leads to a decreased cross-sectional surface area of the pulmonary vascular bed. In addition, underdevelopment pulmonary hypertension is frequently associated with maldevelopment of the pulmonary vasculature (43).
Infants with maldevelopment pulmonary hypertension frequently present with evidence of poor placental function and poor adaptation to their extrauterine environment. Evidence for poor placental function may present as a poorly nourished infant with evidence of fetal weight loss. The perinatal period is often associated with marked fetal distress because labor taxes the function of the compromised placenta. Thick meconium may be noted and the infant may be depressed or asphyxiated at birth. Even without these adverse perinatal events, the abnormal pulmonary vascular bed may not allow the normal rapid initial drop in pulmonary vascular resistance and subsequent increase in pulmonary blood flow which are essential for appropriate cardiopulmonary physiology and adaptation to an adult-type circulation in series with high pulmonary blood flow and air exchange in the lung.
Respiratory Physiology
In the fetus, the organ of respiration is the placenta and the lung is a high resistance, minimal flow, liquid-filled organ. Furthermore, the fetal lung secretes fluid into the airway (44). During late gestation surfactant production increases in preparation for the lung becoming the organ of respiration, and the molecular processes essential for fluid absorption are induced (45). This induction is enhanced by labor. At delivery with the onset of regular respirations, optimal air exchange physiology occurs when lung volume is adequate and pulmonary vascular resistance drops allowing the resultant increase in blood flow.
Disorders of transition occur when any one of the three critical steps do not occur or are delayed. The three disorders of transition are: (a) transient tachypnea of the newborn, which occurs when the removal of lung water is delayed, (b) RDS, formerly known as hyaline membrane disease, and (c) pulmonary hypertension of the newborn which occurs when the normal drop in pulmonary vascular resistance does not occur or is delayed. Each of these disorders has a characteristic physiology which leads to alterations in air exchange. In most cases, the primary aberration is hypoxemia, even though the physiology by which this aberration occurs is different.
In order to understand respiratory physiology of the newborn it is critical to understand some of the physical properties of the lung that determine ventilation. The equation of motion describes the properties of the lung important for proper ventilation:

where P represents the pressure applied to the respiratory system, c is compliance which is defined as the change in volume divided by
the change in pressure, describing the elastance of the respiratory system, V is volume, R is resistance, [V with dot above] is the flow, I is inertance, and [V with dot above] is acceleration. Thus, properties of the lung can be divided into the static properties of the lung which are measured when there is no flow and is dependent on the compliance term in the equation of motion, and the resistive properties of the lung, which are measured when there is flow and are dependent on the resistance term in the equation of motion and inertance which, in most cases is thought to be negligible relative to the static and resistive properties and is therefore ignored. In well newborn infants the compliance is normal and the resistance is low so that minimal effort or energy is needed to provide reasonable ventilation to the respiratory system independent of whether the infant is doing the work or if the infant requires mechanical ventilation.
the change in pressure, describing the elastance of the respiratory system, V is volume, R is resistance, [V with dot above] is the flow, I is inertance, and [V with dot above] is acceleration. Thus, properties of the lung can be divided into the static properties of the lung which are measured when there is no flow and is dependent on the compliance term in the equation of motion, and the resistive properties of the lung, which are measured when there is flow and are dependent on the resistance term in the equation of motion and inertance which, in most cases is thought to be negligible relative to the static and resistive properties and is therefore ignored. In well newborn infants the compliance is normal and the resistance is low so that minimal effort or energy is needed to provide reasonable ventilation to the respiratory system independent of whether the infant is doing the work or if the infant requires mechanical ventilation.
In infants with primary lung disease, the most common biochemical derangement is arterial hypoxemia. The mechanisms for significant hypoxemia in infants with lung disease are primarily ventilation–perfusion abnormalities and/or right-to-left shunting (both intrapulmonary and extrapulmonary). The sum of the shunt fraction and the ventilation–perfusion inequalities is the venous admixture. The venous admixture is higher in newborns even without lung disease (46,47). In parenchymal lung disease, the venous admixture increases dramatically and arterial hypoxemia may become profound. Furthermore, the relative proportion of the shunt fraction and V/Q abnormalities is dynamic such that as ventilation of the lung improves it has been shown that the shunt fraction and low V/Q compartments may be affected independently or in tandem. The primary strategy in infants with lung disease is to improve the function of the low V/Q compartment. Administration of supplemental oxygen may improve the oxygen concentration in the terminal air units and may relieve hypoxic pulmonary vasoconstriction, in which case improvement in oxygenation occurs by decreasing the size of the shunt compartment with no effect on the low V/Q compartment. Improving the ventilation to the low V/Q compartment of the lung frequently addresses both the shunt compartment and the low V/Q compartment. In this case, these strategies may recruit the shunt compartment by improving ventilation, raising the partial pressure of oxygen, and relieving hypoxic pulmonary vasoconstriction. The same strategies may recruit the former low V/Q compartment into the normal V/Q compartment at the same time. Thus while administration of supplemental oxygen is relatively safe and may decrease the shunt compartment, supportive strategies to safely increase ventilation to the most diseased areas of the lung may affect the shunt compartment and low V/Q compartment simultaneously.
The most appropriate strategy to support the respiratory system in the diseased lung depends on the physical properties of the lung, including the static properties and the resistive properties. It is appropriate to bear in mind that supportive measures which improve the function of the diseased lung do not improve the underlying disorder. In fact, supplemental oxygen is toxic and the application of positive pressure to the lung causes injury (48). When positive pressure is administered the strategy utilized should be tailored to the abnormalities in the lung. In lung diseases dominated by low respiratory system compliance, such as RDS or hyaline membrane disease, the airways and airspaces fill and empty quickly so that if mechanical ventilation is utilized, a fast rate-low tidal volume ventilation strategy should be used and has been associated with better outcomes (49). In diseases dominated by high airway resistance, a ventilator strategy using a slower rate with slightly higher tidal volume ventilation is more successful. Examples of such diseases include meconium aspiration and BPD. In infants with RDS, the safest and most efficient technique to improve lung physiology is by increasing the mean airway pressure and the safest and most efficient technique to increase mean airway pressure is to increase the end pressure (50). In fact, many infants with RDS can be managed with CPAP only which is the preferable mode in relatively mature infants (51,52).
Cardiopulmonary Interaction
The pulmonary vasculature in the lung of infants is fully recruited at rest and is particularly predisposed to the development of pulmonary edema when flow is increased via anatomic left-to-right shunts. The rate of fluid filtration (Qf) in the lung or any other organ or vascular bed is governed by the Frank–Starling equation:

where Kf is hydraulic conductance, Pmv is hydrostatic pressure in the microvasculature, Ppmv is hydrostatic pressure in the perimicrovascular space, σ (sigma) is the reflection coefficient, πmv is oncotic pressure in the microvasculature, and πpmv is oncotic pressure in the perimicrovascular space.
Abnormalities in Kf and Pmv are the dominant variables in fluid filtration. Kf is a function of the number and size of the pores in the endothelial cell layer. Disorders that injure endothelial cells markedly increase Kf and fluid filtration (52). When Kf is increased and leads to pulmonary edema, it is characterized as permeability pulmonary edema. There are many disorders that increase Kf and lead to pulmonary edema, but the most common disorders confronted in the newborn period include RDS and sepsis both of which are frequently associated with lung inflammation and a component of lung edema secondary to inflammatory injury (53,54,55). Unfortunately mechanical ventilation has the potential to induce lung inflammation, thus careful supportive care to limit lung inflammation is imperative.
High-pressure pulmonary edema is common in the newborn period, since the lung of the newborn is fully recruited at rest, left-to-right shunts increase fluid filtration substantially (56,57), and an elevation of left atrial pressure also increases filtration. The equation for Pmv is:

where c is equal to a constant between 0 and 1 and PA and LA are pulmonary arterial and left atrial, respectively. This equation illustrates the significant impact of elevated PLA in lung fluid filtration. PLA (or pulmonary venous pressure) can be particularly elevated in some forms of congenital heart disease (e.g., obstructed anomalous pulmonary venous connection, mitral valve disease, or hypoplastic left heart syndrome). Fluid accumulation in the lung can only occur when fluid filtration exceeds lymphatic function which returns filtered fluid to the venous circulation (Qf > QL). QL is dependent on intrinsic contractility of the lymphatics in the lung and on the right atrial pressure. Right atrial pressure may be elevated in infants with lung disease enhancing fluid accumulation in the lung and the pressures at which lymphatic flow is impaired are lower in the fetus and newborn than in older patients. In summary, infants with lung disease and/or heart diseases are more predisposed to develop pulmonary edema than in any other age group because increases in flow are invariably associated with increased microvascular pressure and developmentally impaired lymphatic function is frequently incapable of meeting the demands of increased fluid filtration.
The effects of pulmonary edema on respiratory physiology are dependent on the location of the edema fluid. Typically fluid filtration occurs in the alveolar capillary, but hydrostatic forces in the lung favor fluid accumulation in the extra-alveolar interstitium (58). The extra-alveolar interstitium contains airways so that fluid can compress the airways leading to constriction and increased airway resistance (59). This explains the finding of cardiac asthma and increased airway resistance with pulmonary edema. Decreased respiratory system compliance can also occur with pulmonary edema, primarily when accumulation occurs in the alveolar space and impairs surfactant function. It is difficult to filter fluid to the extent that fluid accumulates in the alveolar space because respiratory
epithelial cells are excellent barriers to fluid egress and they actively pump fluid from the alveolar space into the interstitium (60). The extra-alveolar interstitium can accumulate excessive fluid before it egresses into the alveolar space. However, the lung is more susceptible to alveolar fluid accumulation when increased fluid filtration is associated with injury to respiratory epithelial cells.
epithelial cells are excellent barriers to fluid egress and they actively pump fluid from the alveolar space into the interstitium (60). The extra-alveolar interstitium can accumulate excessive fluid before it egresses into the alveolar space. However, the lung is more susceptible to alveolar fluid accumulation when increased fluid filtration is associated with injury to respiratory epithelial cells.
Treatment for pulmonary edema is almost entirely supportive, and includes measures to lower Pmv or to support the respiratory system by applying positive pressure. Measures to decrease Pmv include reducing pulmonary blood flow by decreasing left-to-right shunts, or by decreasing circulating blood volume. Decreasing fluid administration is a reasonable short-term maneuver to decrease fluid filtration, but only if the fluid restriction does not impair the delivery of adequate nutrition. Therapy with diuretics may also reduce Pmv by decreasing circulating blood volume. While equipotent doses of diuretics improve lung function by decreasing lung water, furosemide has the additional effect of pulmonary dilation (61) which lowers Pmv further. Thus this diuretic is more effective for pulmonary edema than are equipotent doses of other diuretics. Pulmonary edema may accumulate to the extent that pressure support to the lung is necessary. The delivery of positive pressure does not decrease fluid accumulation in the lung. It simply improves the acute physiology, usually by improving V/Q mismatching (62,63) by improving ventilation to the low V/Q compartment in the lung. Decreasing pulmonary edema by decreasing Kf or by improving lymphatic function would be optimal, but measures to consistently achieve these improvements have not been described.
Abnormalities of pulmonary vascular physiology are confined primarily to inappropriate vasoconstriction. Persistent pulmonary hypertension in the newborn occurs when the normal decrease in pulmonary vascular resistance that occurs at birth does not occur and can be a result of underdevelopment, maldevelopment, or maladaptive forms of pulmonary vascular disease as described previously. Pulmonary hypertension in the newborn frequently presents with significant hypoxia because of right-to-left shunting at the fetal shunt pathways at the atrial and ductal levels. Pulmonary constriction is usually on the arterial side of the circuit so that therapies which dilate the pulmonary circuit may improve hypoxemia without increasing fluid filtration if the venous circuit is normal.
Pulmonary hypertension was the most common reason for placing infants on extracorporeal life support because specific pulmonary vasodilators had not been identified. However, inhaled nitric oxide has unequivocally improved the abnormal pulmonary physiology observed in infants with pulmonary hypertension. In several large randomized trials, nitric oxide was effective at reducing the incidence of death or the need for extracorporeal support (64). Inhaled nitric oxide was approved for utilization in infants with hypoxic respiratory failure in 1997 and was restricted to infants at or beyond 34 weeks of gestation. The utilization of this drug has meant that treatments previously used to support these infants, including high concentrations of oxygen and/or induced alkalosis, are contraindicated. Typical indications for the utilization of inhaled nitric oxide in term or near-term infants are respiratory failure as indicated by an oxygenation index of >20 to 25 (OI = FiO2 × mean airway pressure × 100/PaO2) and evidence of pulmonary hypertension from Doppler echocardiography. Supportive efforts with mechanical ventilation and/or administration of surfactant that improve lung inflation improve the efficacy of inhaled nitric oxide (65). Nitric oxide is started at 20 ppm and is decreased in 50% decrements when supplemental oxygen requirements decrease substantially, usually to <50%. When the dose of nitric oxide is 5 ppm, further decrements must be done cautiously as rebound pulmonary hypertension has been described in this range (66). Infants <34 weeks of gestation may also present with hypoxic respiratory failure and recent trials have been published suggesting that these infants may also benefit from treatment with nitric oxide (67,68,69). However, nitric oxide therapy has not yet been approved for these infants.
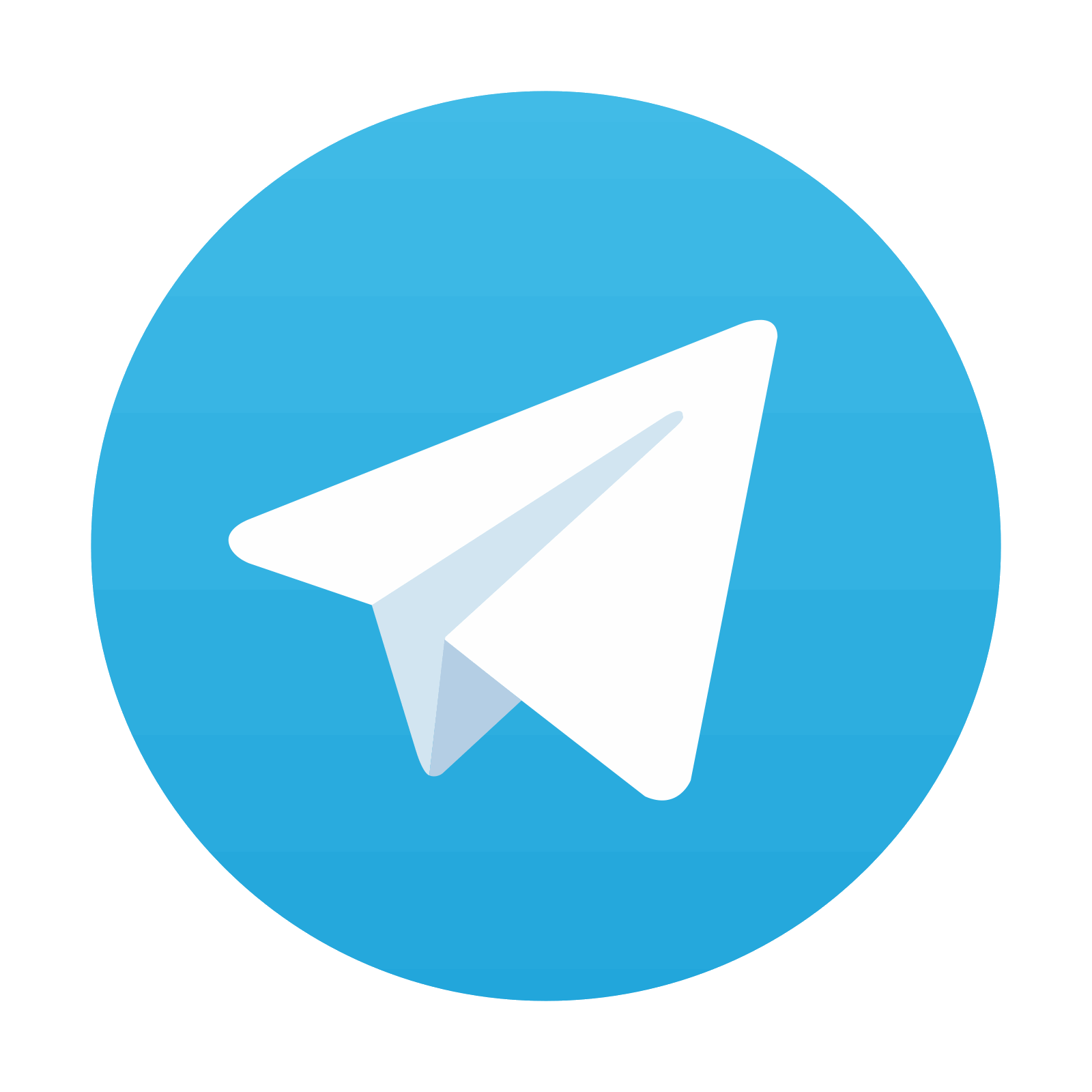
Stay updated, free articles. Join our Telegram channel
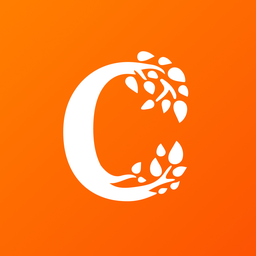
Full access? Get Clinical Tree
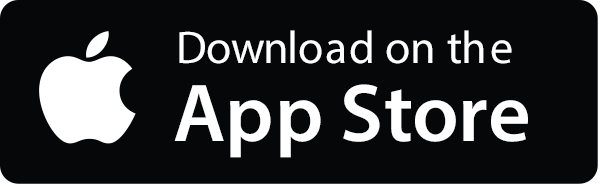
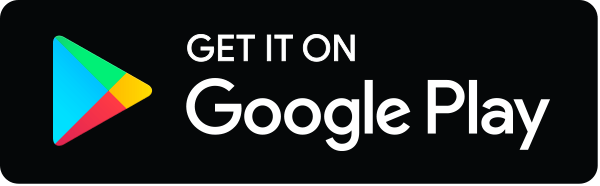