Fig. 4.1
A schematic drawing of the pleuropulmonary compartments involved in the turnover of fluid within the parietal extrapleural interstitium, the pleural space and the pulmonary interstitium. The red arrows indicate the fluid filtration across either the systemic, pulmonary endothelium or parietal mesothelium. The yellow arrow indicates the absorption of pleural fluid towards the parenchyma. The blue arrows represent the absorption of pleural and pulmonary interstitial fluid into the respective lymphatic networks
4.1 Morphology of the Parietal and Visceral Pleural Tissue
4.1.1 Mesothelial Cells
Mesothelial cells are flat, squamous-like cells approximately 1–4 μm thick (Wang 1974) with an average diameter of an estimated 25 μm. They contain few organelles, including microtubules and microfilaments, vesicles and vacuoles, a few mitochondria and poorly developed Golgi apparatus and rough endoplasmic reticulum. Cells are connected by tight junctions, adherens junctions in their apical portion (Fig. 4.2), gap junctions and desmosomes at their basal side; the luminar surface of the cells, especially on the visceral side, presents a well-developed microvilli brush (Bernaudin et al. 1991; Wang 1974, 1985). The renewal rate of mesothelial cells is very low (<0.5 % of cells undergo mitosis at any one time); however, cell proliferation greatly increases after injury to the mesothelial surface or after exposure to inflammatory agents suggesting a role for mesothelial cells in wound healing but also in serosal fibrosis and adhesion formation (Foley-Comer et al. 2002; Mutsaers et al. 2002). In spite of its apparent delicacy, mesothelial cells constitute, together with the submesothelial interstitial tissue layer, a protective barrier against mechanical insult. They also synthesise saturated (primarily dipalmitoylphosphatidylcholine) and unsaturated phospholipids (stearoyl linoleoyl-phosphatidylcholine typically encountered in surfactant lining the alveoli). Unsaturated phospholipids appear functionally more important in the pleural cavity than in the alveolar lining; this is likely due to their specific ability to greatly reduce the coefficient of friction between sliding surfaces compared to saturated phospholipids (Hills et al. 1982, 1992; Mills et al. 2006). Mesothelial cells synthesise hyaluronan (HA) (Wang and Lai-Fook 2000), a large molecular weight (>106 Da) glycosaminoglycan which is a typical constituent of the extracellular tissue fibre matrix and whose most distinctive properties are related to its viscoelastic behaviour. In fact, since its viscosity is inversely related to the shear rate, or the velocity gradient, it has been proposed that hyaluronan, together with graphite-like phospholipids, may play an important role in lubrication of the pleural surfaces (Andrews and Porter 1973). The cells secrete extracellular fibrous matrix macromolecules such as elastin, fibronectin, glycoproteins, proteoglycans and collagen type I, II and IV (Arai et al. 1975; Bernaudin et al. 1991; Rennard et al. 1985). Finally, mesothelial cells secrete various pro-, anti- and immunomodulatory mediators, such as chemokines, cytokines and growth factors, prostaglandins and prostacyclin, reactive nitrogen and oxygen species and antioxidant enzymes, thus playing an important role in the postinflammatory tissue remodelling (Mutsaers et al. 2002).
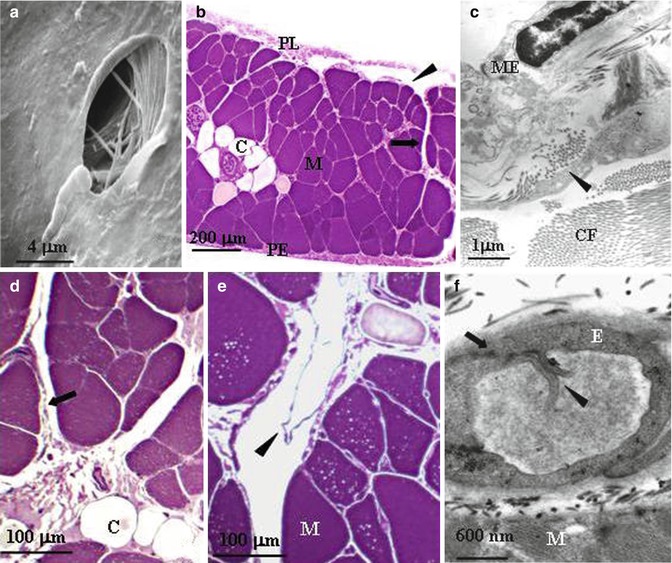
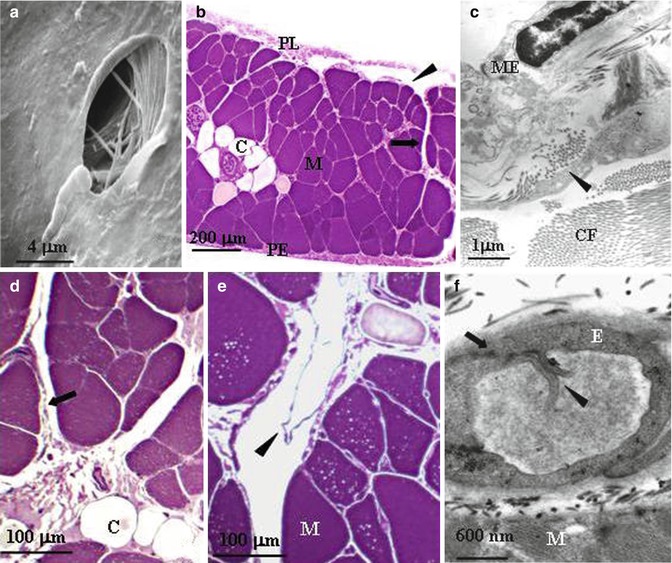
Fig. 4.2
(a) Scanning electron microscope photomicrograph of a lymphatic stoma on the tendinous pleural diaphragmatic surface (Modified from Negrini et al. (1991a, b, c)). (b) Semithin cross section of rat diaphragm showing the arrangement of the diaphragmatic lymphatic network originating from the pleural (PL) and the peritoneal (PE) surfaces. Four types of lymphatic structures may be distinguished: submesothelial lacunae (arrowhead) within the interstitial space beneath the mesothelial layer; transverse ducts (arrow) running perpendicularly to the lacunae through the muscular fibres (M); and central collectors (C), in the deep diaphragm. (c) At transmission electron microscopy (TEM), the pleural submesothelial connective tissue layer is composed of loose collagen fibres (CF) organised in bundles and contains lymphatic lacunae lined by a discontinuous endothelium (arrowheads). ME mesothelium. (d) Transverse ducts (arrow) lined by a discontinuous endothelium and central collector vessels (C) lined by a continuous endothelium. (e) Transverse lymphatic ducts with intraluminar valves (arrowheads) formed by two leaflet attached at opposite sides of the lymphatic vessel walls. (f) Primary valves (arrowheads) formed by two adjacent endothelial cells of a diaphragmatic initial lymphatic (arrow) (b, c, d, e, f modified from Grimaldi et al. (2006))
4.1.2 Subpleural Interstitial Space and Blood Supply
Mesothelial cells rest on a thin basement membrane supported by a layer of submesothelial interstitial space with a thickness of approximately 10–20 μm on the visceral pulmonary surface and 100–150 μm on the parietal side (Albertine et al. 1982, 1984; Mariassy and Wheeldon 1983). In all mammalian species, the parietal submesothelial interstitium is supplied by blood capillaries arising from the systemic circulation. In contrast, the blood supply to the visceral submesothelial interstitium may derive either from the bronchial (as in human, sheep, horse and pig) or the pulmonary (as in dog, cat and rabbit) circulation (Agostoni 1986).
4.1.3 The Pleural Lymphatic System
An important and unique feature of the parietal pleura which is not shared by the visceral mesothelium is the presence of so-called stomata (Fig. 4.2a). These are discontinuities of the parietal mesothelium and of the submesothelial interstitial space which form cylindrical-like openings creating the origin of the pleural lymphatic system (Negrini et al. 1991b; Wang 1975). Although widely spread over the diaphragmatic, costal (average density, ~100/cm2) and mediastinal parietal pleura, the greater density is encountered on the diaphragmatic surface (~8,000/cm2), where they are distributed on the tendinous and the muscular region of both the pleural and peritoneal surfaces (Negrini et al. 1991a). The stomata (the average diameter ranges from 0.5 to 20 μm) form at the confluence between the mesothelial cells of the parietal pleura and the lymphatic endothelial cells and empty into an extensive network of lymphatic submesothelial lacunae (Grimaldi et al. 2006; Negrini et al. 1992a), which is located within the interstitial space beneath the mesothelial monolayer (Fig. 4.2b). On the diaphragm, the lymphatic lacunae and the lymphatic capillaries originate directly from the interstitial tissue located amongst the skeletal muscle fibres (Fig. 4.2c) and empty into a system of transverse lymphatic ducts, which depart perpendicularly from the submesothelial lacunae and in turn connect to the deep central collecting system located in the deep interstitial space and surrounded by a continuous endothelial layer (Fig. 4.2d, e). All vessels belonging to this complex network share the same ultrastructural features typical of the so-called initial lymphatic vessels: (a) the basal lamina is discontinuous, (b) anchoring filaments of collagen 3 VII (Schmid-Schöenbein 1990) tightly and directly bond the outer surface of the lymphatic endothelial cells to the fibrous components of the extracellular tissue matrix connective tissue or to muscle fibres and (c) endothelial cells are joined by tight or overlapping junctions. The structure of the lacunae wall, delineated by a discontinuous endothelium and apparently devoid of smooth muscle cells, suggests that these structures do not possess the spontaneous vasomotion that characterises, for instance, the lymphatic mesenteric vessels (Benoit et al. 1989; Zawieja et al. 1993). Initial lymphatic vessels are equipped with two types of unidirectional valves: primary valves in the vessel wall, formed by cytoplasmic extensions of adjacent endothelial cells protruding into the capillary lumen (Fig. 4.2f); this regulates fluid and solute entrance from the interstitial space into the lymphatic lumen. In addition, the intraluminar valves, formed by a leaflet protruding from the lymphatic vessel wall (Fig. 4.2e), direct lymph through the vessel network towards the larger collecting lymphatics. The transverse lymphatic ducts and the central collecting system receive the newly formed lymph from the pleural submesothelial lacunae and from the internal and deeper interstitium and function as a collecting system for the lymph to be carried out of the diaphragm through extra-diaphragmatic collectors, mostly through the right and, to a lesser extent, the left lymphatic ducts.
The pleural lymphatic network is not limited to the diaphragm, but extends to the intercostal and to mediastinal tissues where stomata have also been described (Wang 1975). Conversely, no evidence of stomata has been reported for the visceral pleura, which is supplied by the pulmonary lymphatic network (Albertine et al. 1982; Hainis et al. 1994). Therefore, the lymphatic drainage of the pleural space and of the lung parenchyma occurs through completely independent networks.
4.2 Pleural Surface Pressure
The importance of the pleural space in terms of respiratory physiology depends on two elastic structures: the lung and the chest wall. Like all elastic structures, the lung and the chest tend to passively assume their resting volume, corresponding to the position of minimal mechanical energy. The two structures have different shapes and mechanical properties—the resting volume of the chest is much larger compared to the lung. Therefore, to attain their respective mechanical equilibrium, the chest tends to expand while the lung tends to collapse. The recoil force of the lung and the chest wall per unit pleural surface area, defined as pleural surface pressure (P pl), tends to pull apart the two structures, lowering the pressure in the pleural space (intrapleural depression). As a consequence of the effect of gravity on the lung tissue, lung recoil is not uniform, but varies with lung height: in humans, at a volume corresponding to the functional residual capacity (FRC), the average P pl is ~−6 cm H2O at the heart level and decreases by approximately 0.2 cm H2O/cm with increasing lung height (Agostoni 1972, 1986). The P pl gravity distribution is extremely important from the mechanical standpoint, in that it determines local transpulmonary pressure and regional lung expansion (Agostoni 1986; Lai Fook 2004).
4.3 Pleural Fluid Volume and Composition
The mammalian pleural space contains fluid, whose volume (V pl) decreases with body mass, ranging from ~2.5 ml/kg in rats to ~0.04 ml/kg in pigs (Miserocchi et al. 1984), and is 0.26 ml/kg in healthy human subjects (Noppen et al. 2000). The fluid is distributed on an average total pleural surface area of ~90 cm2/kg of body weight (Miserocchi and Agostoni 1971). Pleural fluid thickness is also very variable amongst different species and within the same species in various regions of the pleural space, ranging between 5 μm over the flat costal surface and 60–100 μm in fluid pools collecting at the lobar margins, at the lung base and in the diaphragmatic apposition zones (Lai-Fook and Kaplowitz 1985). The presence of freely moving fluid into the pleural space is common to all mammals, with the exception of the elephant, whose pleural space is liquid filled only in foetal life, and becomes obliterated by connective tissue at birth and remains obliterated during the animal’s entire life (West 2002).
Although the ionic composition of pleural fluid mirrors that of the extracellular extravascular fluid compartment, its physiological protein concentration (C pr) is smaller compared to other interstitial spaces, varying between 2.5 g/dl in rats and 1.2 g/dl in pigs (Miserocchi et al. 1984). The normal concentration of glucose and lactic dehydrogenase (LDH) in pleural fluid is similar or slightly smaller (pleural fluid to serum concentration ratio ~0.9) than the corresponding serum levels (Sahn and Light 1989). However, these parameters usually increase during inflammation when, associated to decreased pleural fluid pH, they are considered as some of the most important clinical indicators to distinguish between transudative and exudative pleural effusions (Joseph et al. 2001; Sahn and Light 1989).
The pleural fluid also contains saturated and unsaturated phospholipids and hyaluronan, derived from mesothelial cells. While significant levels of HA are found in lung tissue and in the mesothelial microvilli layer (Fraser and Laurent 1996), the normal pleural fluid hyaluronan content (~0.7 μg/ml) (Allen et al. 1992) is not considered significant to contribute to the lubrication of the mesothelial layer. HA dramatically increases in malignant pleural effusions (Thylén et al. 2001) and indeed is a significant prognostic value in malignant mesothelioma. Various cell types, such as detached mesothelial cells, lymphocytes, monocytes and macrophages, are dispersed in the normal pleural fluid at a concentration of 1,500–2,500 cells/mm3 (Miserocchi and Agostoni 1971; Noppen et al. 2000). However, the concentration and type of cells in pleural fluid does change in several mesothelial and pulmonary pathologies, offering important prognostic tool to differentiate between the various diseases.
4.4 Pleural Fluid Turnover
A direct consequence of the mechanical arrangement of the lung and chest wall and of the existence of the intrapleural depression is that fluid is continuously driven across the mesothelium into the pleural space (see Sect. 4.3); this could potentially lead to a progressive accumulation of pleural fluid and to lung collapse. Therefore, in order to maintain the tight mechanical lung–chest wall coupling, pleural fluid must be continuously removed from the pleural space.
In normal conditions, filtration and corresponding removal of pleural liquid would amount to 0.02–0.09 ml/(h kg body weight) (Miniati et al. 1988; Negrini et al. 1985). Pleural fluid production and its subsequent removal from the pleural space occur through different mechanisms involving not only the pleural mesothelial lining but also in the other compartments schematized in Fig. 4.1.
4.4.1 Filtration of Fluid into the Pleural Space
Fluid and soluble solutes may cross biological barriers, such as the capillary endothelium or the mesothelial layer, through either paracellular pathways between adjacent cells or through vesicular transport (transcytosis) across the cell itself.
4.4.1.1 The Paracellular Transport
Paracellular transport is a passive passage of water and hydrophilic solutes through the intercellular clefts between adjacent cells. The fluid bulk flow (J v) between any two compartments a and b is set by the hydraulic (P) and colloid osmotic (π) pressure differences across the membrane as described by Starling’s law:
![$$ \begin{array}{c}{J}_{\text{v}}={L}_{\text{p}}\cdot {A}_{\text{m}}\cdot [({P}_{a}-{P}_{b})\\ -\sigma \cdot ({\pi }_{a}-{\pi }_{b})]={L}_{\text{p}}\cdot {A}_{\text{m}}\cdot \Delta P\end{array}$$](/wp-content/uploads/2017/03/A191147_1_En_4_Chapter_Equ00041.gif)
where L p is the hydraulic conductivity of the membrane, A m is the surface area, σ the reflection coefficient of the membrane to plasma proteins and ΔP the net transmembrane pressure gradient.
![$$ \begin{array}{c}{J}_{\text{v}}={L}_{\text{p}}\cdot {A}_{\text{m}}\cdot [({P}_{a}-{P}_{b})\\ -\sigma \cdot ({\pi }_{a}-{\pi }_{b})]={L}_{\text{p}}\cdot {A}_{\text{m}}\cdot \Delta P\end{array}$$](/wp-content/uploads/2017/03/A191147_1_En_4_Chapter_Equ00041.gif)
(4.1)
This equation was derived (Curry and Frøkjaer-Jensen 1984) by viewing the transmembrane fluid fluxes as viscous laminar flows through n parallel intercellular pores of equal radius (r) and length (l), where
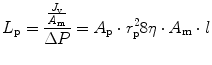
A p and η are the total area of pores and fluid viscosity, respectively, and σ is a pure number (0 < σ < 1) which in turn depends on the ratio of the radius of a given solute to the radius of the intercellular porous and provides an index of the capability of the solute to cross the intercellular porosity of the membrane. Ions and smallest solutes (σ → 0) freely cross the endothelial clefts and equilibrate across both the vascular endothelium and the mesothelium, without providing any osmotic contribution to fluid flux. Vice versa, large molecular weight plasma proteins are restricted in their movement across either the capillary endothelium (σ ~ 0.8) or mesothelium (σ ~ 0.2–0.4) (Negrini et al. 1990, 1991c), generating a colloid osmotic pressure gradient across any two membranes.
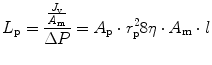
(4.2)
A very important parameter appearing in Eq. 4.1 is the hydraulic pressure in compartments a and b or, when examining pleural fluid exchange, in pleuropulmonary compartments described in Fig. 4.1.
Pleural liquid pressure (P liq) has been extensively measured through fluid-filled cannulae and/or micropipettes inserted in various regions of the intact pleural space and at various lung heights in experimental animals (Miserocchi and Agostoni 1980; Miserocchi et al. 1981; Miserocchi et al. 1988). In all pleural regions (costal, diaphragmatic and mediastinal) and at any lung height, P liq is lower than P pl—both at end-expiration (Fig. 4.3a) and at end-inspiration (Fig. 4.3b). This indicates that the mechanism providing pleural fluid removal from the pleural space is capable to decrease the intrapleural pressure below the values set by lung recoil. In addition, because of the significant pressure variability encountered within the space, pleural fluid continuously flows from the costal to the extracostal (diaphragmatic and mediastinal) regions and within each region in a gravity-dependent fashion (Fig. 4.4b). Extensive measurements performed in experimental animals have shown that the pressures in the interstitial space below the parietal mesothelium (parietal extrapleural interstitial pressure, P epl) and the visceral mesothelium (pulmonary interstitial pressure, P pi) are also subatmospheric at any lung height (Fig. 4.3a). In particular, P pi is always more subatmospheric than P liq, approximately −10 cm H2O at heart level and FRC and −22 cm H2O at end-inspiration (Miserocchi et al. 1990, 1991). According to Eq. 4.1, the flow direction across a membrane is set by ΔP direction: therefore, from the hydraulic (Miserocchi et al. 1984; Negrini et al. 1993; Negrini and Miserocchi 1989) and colloid osmotic (Negrini et al. 2001) pressure values measured in the pleuropulmonary compartments, we may calculate ΔP across the mesothelial and endothelial membranes (Fig. 4.1). Data indicates that fluid filtration into the pleural space depends upon fluid transfer from the systemic capillaries into the parietal extrapleural interstitial space and from the latter into the pleural space through the parietal mesothelium (Figs. 4.1 and 4.4a). As clearly indicated by Eq. 4.1, the absolute pleural filtration flow per unit surface area is modulated by the L p of the filtering membranes. In spite of what is commonly reported in studies performed on oedematous or isolated lungs, or on stripped parietal or visceral pleura which suggests that both pleural mesothelia are very permeable to water and even proteins, the L p of the normal, in situ parietal pleura is relatively low (Negrini et al. 1990, 1991c, 1994) and comparable to that of the endothelium of the systemic capillaries of skeletal muscles (Rippe and Haraldsson 1994; Taylor and Parker 1985). The molecular sieve and the viscous flow resistance of the passive paracellular pathway are likely provided by the fibrous interstitial matrix in the intercellular cleft and by the endothelial cell glycocalyx (Adamson and Michel 1993). The complexity of junctional strands accounts for the fact that less than 10 % of the cell-to-cell contact area is available for microvascular transport and this represents less than 0.04 % of the total capillary surface area (Michel and Curry 1999). At variance with what is observed for the parietal pleura, in normal conditions, the lung parenchyma does not participate to the formation of pleural fluid.
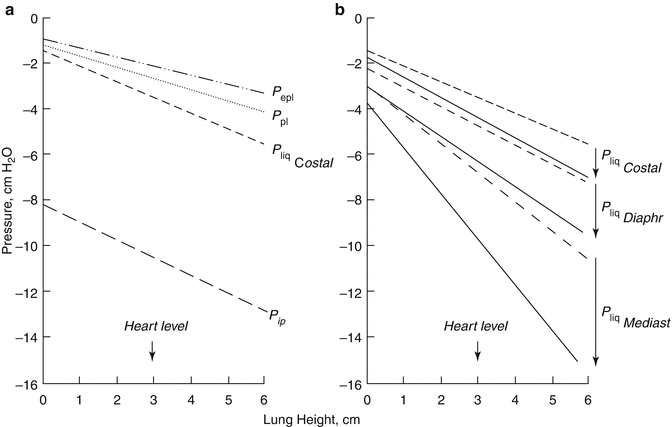
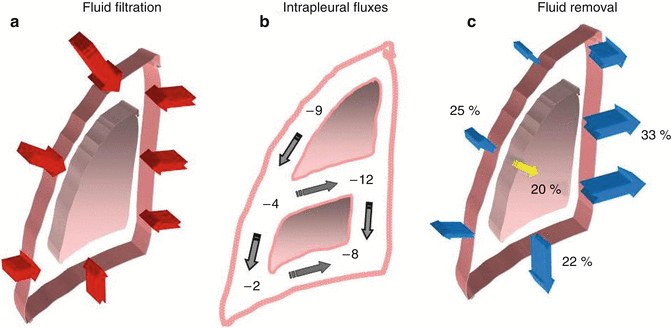
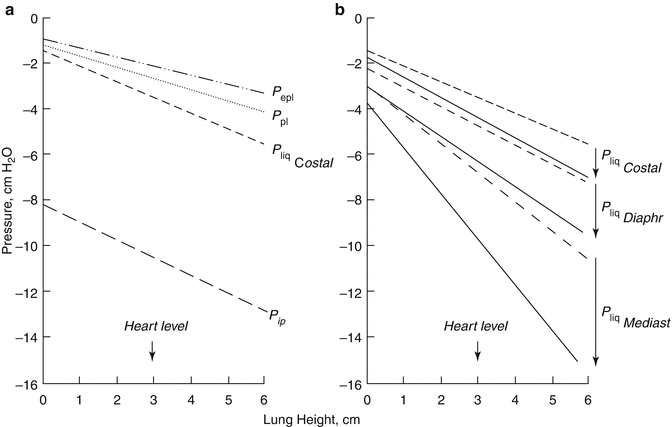
Fig. 4.3
(a) The hydraulic pressures in the costal region of the pleural space (P liq, Miserocchi et al. 1983) and in the parietal extrapleural (P epl, Negrini et al. 1987) and pulmonary (P pi Miserocchi et al. 1993) interstitial spaces as a function of lung height in supine anaesthetised rabbits and at end-expiratory lung volume (FRC). The pleural surface pressure, an index of region lung recoil, is also reported for comparison (dotted line, Agostoni 1986). (b) The costal, mediastinal and diaphragmatic P liq values at the end-expiratory (dashes lines) and end-inspiratory (continuous lines) lung volumes (Miserocchi et al. 1981)
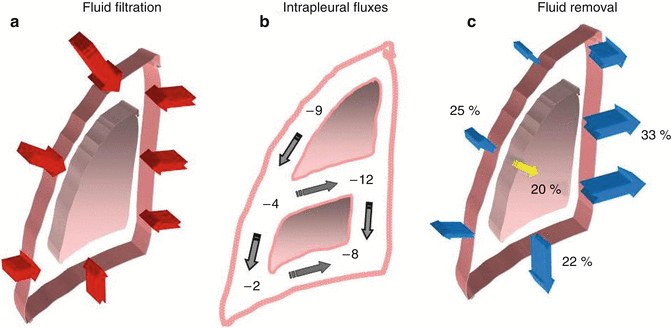
Fig. 4.4
(a) The sites of preferential pleural fluid filtration through the parietal mesothelium. (b) The top to bottom and costal to extracostal intrapleural flow direction. (c) The sites of preferential removal of pleural fluid into the costal, mediastinal and diaphragmatic pleural lymphatic system
4.4.1.2 Transcytosis
Transcytosis is an energy-dependent process consisting of the transport of water and solutes across the plasmalemma and cytoplasm through small grouped vesicles called caveolae (Bruns and Palade 1968). Transcytosis may consist of endocytosis of a small quantity of fluid followed by transcellular transport or may require recognition of the target macromolecule at the membrane surface through a receptor-mediated mechanism. In the pulmonary endothelium, transcytosis has been described in the transport of albumin, fatty acids and hormones (Schnitzer and Oh 1996; Schnitzer et al. 1995). The process is completely independent upon pressure gradients; therefore, transcytosis might, in principle at least, provide fluid movements either from the extrapleural interstitial space of both the parietal and visceral pleura into the pleural space, thus contributing to fluid filtration, or in the opposite direction, contributing to pleural fluid removal from the pleural space into the adjacent visceral and parietal interstitial space. Data obtained from stripped isolated pleura or isolated pericardium (Bodega et al. 2002) estimates that transcytosis would contribute to approximately 5 % of the interstitial-to-pleural fluid exchange across both mesothelial layers (Agostoni and Zocchi 2007).
4.4.2 Removal of Fluid from the Pleural Space
While pleural fluid production almost completely depends upon passive transmembrane transport across the parietal pleura (Sect. 4.1), fluid egress from the pleural cavity may take place, in normal conditions, through a number of different pathways. These include drainage into the pleural lymphatic system, passive transmesothelial flow, solute-coupled intracellular fluid absorption, intracellular transport through aquaporins and transcytosis.
4.4.2.1 Pleural Lymphatic Drainage
Liquid and solutes enter the pleural lymphatic system through the stomata (Fig. 4.2a) which, as previously discussed, are disseminated throughout the costal, mediastinal and diaphragmatic parietal pleura. While the general principles of lymph formation and accumulation are common to all tissues supplied by the lymphatic system (i.e. all body tissues except the cerebral interstitium and renal medulla), however, stomata are typical of the pleural and peritoneal parietal mesothelia. Entrance of fluid and solutes into the stomata occurs along hydraulic pressure gradients (ΔP lymph-net) between the pleural space and the lumen of submesothelial lymphatic lacunae when intraluminar lymphatic pressure (P lymph) drops below P liq, as described by the equation
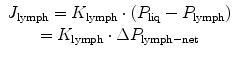
where J lymph and K lymph represents pleural lymph flow and lymphatic conductance, respectively.
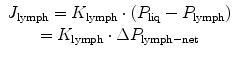
(4.3)
The existence, in initial lymphatics, of a lower pressure regime compared to the surrounding compartment is the necessary prerequisite for lymphatic drainage to take place. In non-thoracic tissues, like the mesentery, lymph formation and propulsion is sustained by spontaneous cyclic contractions of a well-organised layer of smooth muscle cells surrounding the lymphatic endothelium (Aukland and Reed 1993). Contractions of the walls of adjacent lymphatic segments, in association with the synchronised opening/closure of the unidirectional leaflets in the lymphatic vessel wall and in the lumen (Bridenbaugh et al. 2003), allow both lymph formation and its subsequent propulsion out of the tissue and towards the venous system. This circumferential support of smooth muscle cells is almost completely absent in the initial lymphatic vessels supplying the intercostal muscles (Moriondo et al. 2006) and the diaphragm (Grimaldi et al. 2006). Therefore, in thoracic tissues and in the pleural space, lymph formation and progression depends upon cyclic expansion and compression of the initial lymphatic vessels which, in turn, is dependent on tissue movements during the cardiac and/or respiratory cycles (Moriondo et al. 2006; Negrini et al. 2004). A similar mechanism, based on extrinsic cardiogenic and respiratory tissue displacement, likely supports the pulmonary lymph flow.
The ability of the pleural lymphatics to drain fluid was proposed more than a century ago by Sir Ernest Starling (Starling and Tubby 1894). However, until recently, in spite of numerous direct and indirect observations in favour of lymphatic function (Allen and Vogt 1937, Bettendorf 1979; Courtice and Simmonds 1954; Miniati et al. 1988, Miserocchi et al. 1982, 1983, 1984; Miserocchi and Negrini 1986; Negrini et al. 1991a; Negrini and Miserocchi 1989), the physiology of lymphatic drainage of the pleural fluid remained controversial. In fact, lymphatics were considered unable to absorb liquid from a compartment set at subatmospheric pressure and instead it was thought to actively absorb proteins but not smaller solutes and water (Agostoni 1972, Agostoni 1986; Staub et al. 1985; Wiener-Kronish et al. 1984). The definite proof of the ability of pleural lymphatics to generate subatmospheric pressures and to provide an important contribution to pleural fluid drainage came from a series of studies in which P lymph was measured directly in rodent diaphragmatic lymphatics (Negrini and Del Fabbro 1999; Negrini et al. 2004) and in intercostal lymphatics (Moriondo et al. 2006) during spontaneous breathing (Fig. 4.5). Results from these experiments have indicated that lymphatic function in the thoracic tissues and in the pleural cavity depends upon the development of local cyclic tissue stresses associated with the respiratory and cardiac activities that cause P lymph to cyclically oscillate (Fig. 4.5) from values as low as −20 cm H2O to positive pressure (max P lymph +10 cm H2O). The three-dimensional architecture of matrix macromolecules, their arrangement in the intercostal spaces and their mechanical properties seem to be a determinant in transmitting local tissue stress and in turn play a fundamental role in sustaining and modulating lymph formation in the thoracic tissues and in the pleural space. During spontaneous breathing, based on the recorded P liq and P lymph values, the driving pressure gradient (ΔP lymph-net) promoting pleural fluid entrance into the pleural lymphatics ranges between ≈−0.5 cm H2O at end-expiration (Negrini and Del Fabbro 1999; Negrini et al. 2004) and ≈−24 cm H2O at end-inspiration (Moriondo et al. 2006), suggesting that removal of pleural fluid occurs throughout the whole respiratory cycle. The P lymph waves attributable to spontaneous contraction of lymphatic smooth muscle cells, commonly observed in dermal or mesenteric lymphatics, are extremely rare in pleural lymphatics, which behave more like those supplying skeletal muscle (Skalak et al. 1984; Trzewik et al. 2001) and the myocardium (Melhorn et al. 1995), i.e. tissues which undergo high tissue stresses. During spontaneous breathing, the entity of tissue stress, and thus the depth of the inspiratory phase, seems to be more effective than respiratory frequency in favouring pleural lymphatic drainage (Moriondo et al. 2006). After the fluid has drained from the pleural space, the progression of the newly formed pleural lymph towards larger collecting lymphatic vessels is facilitated by the existence of primary unidirectional flap-like valves (Fig. 4.2e, f) which prevent fluid backflow (Grimaldi et al. 2006). Interestingly, during mechanical ventilation at zero end-expiratory alveolar pressure with muscular paralysis, the ΔP lymph-net integrated over the entire respiratory cycle in intercostal lymphatics was essentially nullified (Moriondo et al. 2006), suggesting that active contraction of inspiratory muscles and not simply chest wall expansion is required to enhance lymph formation and progression in thoracic lymphatics. Therefore, because of the importance of the lymphatic system in controlling fluid homeostasis in the thoracic tissues and in particular in the pleural cavity, mechanical ventilation “per se” is expected to determine thoracic tissues overhydration, pleural effusion and, very likely, development of latent pulmonary sub-oedematous conditions in lungs of patients exposed to positive pressure ventilatory regimes.
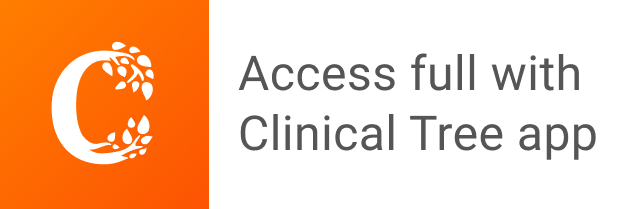