Photo of a child having infant lung function testing. The child has been sedated with chloral hydrate and has had a mask with pneumotachograph attached to their face using putty to prevent leaks.
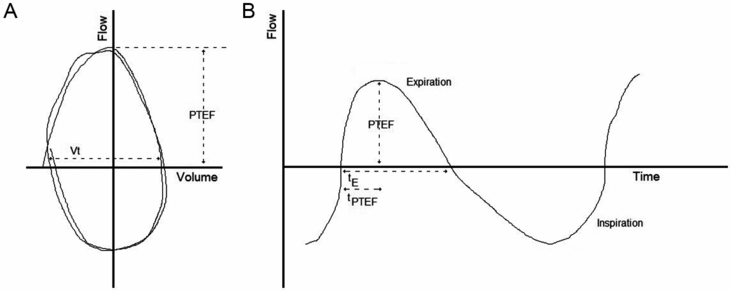
Tidal breathing flow-volume traces. Panel (A) shows tidal volume as flow versus volume. Panel (B) represents tidal volume as flow versus time. PTEF: peak tidal expiratory flow, tE: expiratory time, tPTEF time to reach PTEF.
Detailed information on recommendations for tidal breathing testing and analysis are provided in a document published by the ERS/ATS task force on standards for infant respiratory function testing (3). This paper provides recommendations about software and equipment requirements when analyzing tidal breathing measurements in infants. The guidelines also cover terminology and definitions, equipment, data acquisition and analysis, and reporting of tidal breathing results and highlight areas in which further research is needed before consensus can be reached. There have been many efforts to establish normal reference data for tidal volume variables in healthy infants, with varying success, and therefore the physiological implications and the clinical or diagnostic value of most tidal breathing measurements remain limited (1).
Forced Expiratory Flow-Volume Measurements
In older children and adults who are able to actively cooperate, forced expiratory flows, such as those measured by spirometry, may be used for the evaluation, monitoring, and management of respiratory diseases. By altering the techniques and modifying the equipment a version of these tests can be carried out in infants and preschool children. Forced expiratory flows can be measured in infants using a flow meter attached to a face mask and performing either forced deflation or rapid thoracoabdominal compression (RTC; a squeeze) from tidal or raised lung volumes. Forced deflation is relatively invasive and requires the infant to be intubated and a negative pressure applied, which is why it is not routinely used in a clinical setting (4). The RTC technique requires the application of external pressure, or a “squeeze,” to the thoracoabdominal area and is usually accomplished by wrapping the infant with an inflatable jacket or vest. Rapid inflation of the jacket or vest results in a rapid thoracoabdominal squeeze to empty the infant’s lungs. These measurements are taken during the normal tidal breathing cycle and therefore are only able to describe forced expiratory flows during the limited tidal volume.
The most commonly reported measurement from the RTC is maximal expiratory flow at functional residual capacity, V’maxFRC (Figure 14-3). The shape of the peak expiratory curve also gives an indication of airway function. As with spirometry, a convex curve generally reflects normal airway caliber whereas a concave curve may indicate airway obstruction. Despite several attempts by different researchers, there are still no widely accepted normal reference data available, which is related to differing equipment, ethnicity, socioeconomic, and age-related factors (4).
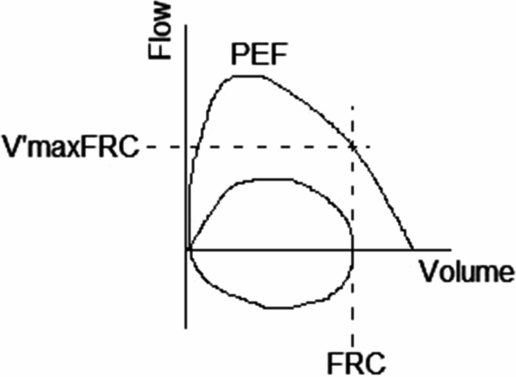
V’maxFRC flow-volume trace. PEF: Peak expiratory flow; FRC: Functional residual capacity; V’maxFRC: Maximal flow rate at FRC.
Another RTC technique involves augmenting the infant’s breath during inspiration to a set inspiratory inflation pressure, thus increasing the breath above normal tidal volumes prior to the rapid squeeze; this technique is called raised volume rapid thoracoabdominal compression (RVRTC). The RVRTC provides expiratory flow information over a greater volume, from near total lung capacity to near residual volume and for this reason is sometimes referred to as infant spirometry. The jacket pressure, or amount of squeeze applied is incrementally increased to a point where further increases do not result in higher flow rates, that is, flow limitation is achieved.
The most commonly reported measurements are similar to those from traditional spirometry and include forced vital capacity (FVC) and forced expiratory volumes at 0.5, 0.75, and 1 s during the forced expiration (FEV0.5, FEV0.75, and FEV1, respectively). Calculation of FEV1 and FVC are not usually feasible, as expiratory times are too short in the infants. Measurements representing forced expiratory flow are also reported and include forced expiratory flow at 50, 75, and 85% of FVC (FEF50, FEF75, and FEF85, respectively). As with spirometry performed in older children and adults, the volume-time measurements are more reproducible than the flow-volume measurements. As with the tidal volume RTC technique, the shape of the forced expiratory flow-volume curve provides information on potential airway obstruction (Figure 14-4). Detailed information on recommendations for RVRTC measurement and analysis are provided in a document published by the ERS/ATS task force on standards for infant respiratory function testing (5).
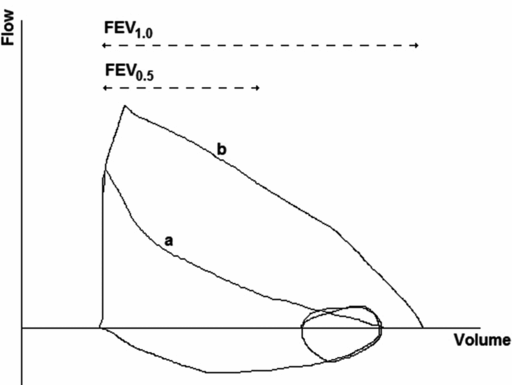
RVRTC flow-volume trace. FEV1.0: Forced expiratory flow at 1 second during a forced expiration; FEV0.5: Forced expiratory flow at 0.5 seconds during a forced expiration. Loop a demonstrates lower inspiratory and jacket (squeeze) pressures; loop b demonstrates higher inspiratory and jacket pressures, nearing flow limitation.
Infant Whole-Body Plethysmography
Quantifying lung volume is important for assessing overall lung growth and development throughout infancy and childhood. Most of the other infant lung function tests are volume dependent, for example, if lung volumes are lower, then FEV0.5 may be lower. The only static lung volume measured during infant lung function is FRC. FRC represents the amount of air left within the lungs at the end of a normal tidal breath, and it includes residual volume. FRC can be measured by whole-body plethysmography (FRCpleth) or by an inert gas washout technique. Infant whole-body plethysmography equipment is available commercially, potentially allowing widespread application of the technique. Whole-body plethysmography requires the infant to lie in a closed, rigid clear container; the plethysmograph. The lid of the container is designed so that the infant can be monitored through the test and is easily accessible if necessary. Within the container a flow meter is attached to the infant, usually via face mask (Figure 14-5).
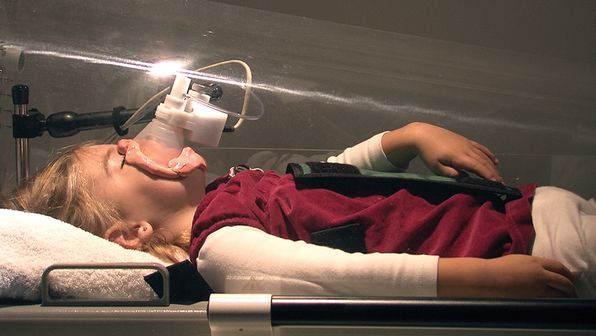
A photo of a child within a whole-body plethysmograph, attached to the pneumotachograph via a face mask.
FRC measured in the whole-body plethysmograph is based on Boyle’s law, which states that with constant temperature the product of pressure and volume of a fixed mass of gas will be constant (4,6). During testing the flow meter is occluded briefly, during which time the infant makes respiratory efforts against the shutter, which compresses and expands the gas within the remaining lung volume, that is, FRCpleth (4,6). The change in alveolar pressure, measured at the mouth or airway opening, is assumed to represent alveolar volume, which reflects changes in the box pressure within the plethysmograph, and is used to calculate lung volume during the occlusion (4, 6). An abnormally elevated FRCpleth may be due to hyperinflation, and abnormally low values may indicate restriction. Airway resistance (Raw) is also measured during this test and is the pressure difference that must be applied between alveoli and the external atmosphere to produce a gas flow of 1 L.s–1 at the airway opening (4,6). The advantage of plethysmographic assessment of Raw is that it can be measured throughout the respiratory cycle and thus reflect dynamic conditions (4,6). Several other variables can be derived from these measurements: airway conductance (Gaw) is the reciprocal of Raw; specific resistance (sRaw) is the product of Raw and FRC (sRaw = Raw.FRC) and can be determined from tidal breathing without the need for airway occlusions (4,6). A standardization document discussing plethysmographic measurements of lung volume and airway resistance in infants was published in 2000 as a result of a joint task force of the ERS/ATS and details recommendations relating to equipment requirements, study procedures, and reporting of data for plethysmographic measurements in infants (7).
Measurement of Functional Residual Capacity and Ventilation Inhomogeneity by Gas Dilution Techniques
Measurement of ventilation inhomogeneity is a rapidly growing area in pediatric respiratory physiology. An essential requirement for effective ventilation is the efficient mixing of inspired gas with the resident gas within the lungs. If this gas mixing is inhomogeneous or inefficient, ventilation will change to ensure adequate gas exchange. The airways change with descent through many divisions from the initial, larger, more proximal conducting zones where gas flow is achieved by conduction (airway generations 1–16), down to areas of the lungs where gas movement occurs by diffusion, the smaller more peripheral acinar zone, where gas exchange occurs. Lung structure, especially in the periphery, airway resistance, and lung compliance, all influence gas-mixing efficiency. Even healthy lungs will have ventilation inhomogeneity. In a normal lung, therefore, some degree of ventilation inefficiency will be present, but if there is airway obstruction, either generalized or in a particular region, but especially in the peripheral airways, the distribution of ventilation will be uneven, with a reduction in gas mixing efficiency and gas trapping. This may be the case in infants with cystic fibrosis (2). The multiple breath washout (MBW) tests assess the efficiency of gas distribution and mixing within the lungs.
Commercial systems are available that measure inert gas washout or gas dilution and can be used in infants. Newer methods are able to measure breath-by-breath gas concentrations and allow for more complex analyses that provide data on overall gas mixing efficiency within the lungs, that is, the inhomogeneity of ventilation (8). The more time it takes to wash-in or wash-out an inert gas, the less efficient is the ventilation. These tests are noninvasive and are performed during tidal breathing.
The most commonly reported variable is the lung clearance index (LCI). The LCI is calculated as the cumulative expired volume needed to lower end-tidal marker gas concentration to 1/40th of the starting concentration divided by FRC, that is, the number of lung volume turnovers needed to clear the marker gas from the lungs. A technique called moment analysis can be used to quantify the degree of inhomogeneity of ventilation distribution as described by the inert gas washout curve. Moment ratios describe the area under the gas washout curve. The higher the moment ratios, the more skewed is the washout curve, which indicates that a greater portion of the lungs is slowly ventilated. Consequently, the LCI will become higher as the lungs must be ventilated for a longer time, and the moment ratios become higher as more of the marker gas leaves the lung late during the washout. Although these simple tests are highly sensitive to airway pathology, particularly obstruction of the peripheral airways, they do not give any information about the mechanisms behind inhomogeneity or where along the airway tree obstruction has occurred.
More complex analysis using the slopes generated during normal tidal breathing suggests that MBW may allow the area of ventilation inefficiency to be localized within the conducting airways (Scond), or within the acinar or gas exchanging zone of the lungs (Sacin). There is a zone within the lungs where ventilation by convection and ventilation by diffusion meet; this zone is known as the diffusion–convection front. Different disease processes occurring within the lungs may move this diffusion–convection front peripherally or proximally and thus alter the Scond and Sacin dependent on where the disease is affecting ventilation efficiency. Phase III slopes are a plot of a single breath measured during the MBW test and show the expired volume of the breath along the x-axis and the concentration of expired gas as the expiration of that breath continues, in this case nitrogen concentration (Figures 14-6 and 14-7). There are different phases throughout the expired breath; first a very low expired gas concentration (phase I), then a rapid rise (phase II), followed by a plateau in the expired gas concentration (phase III).
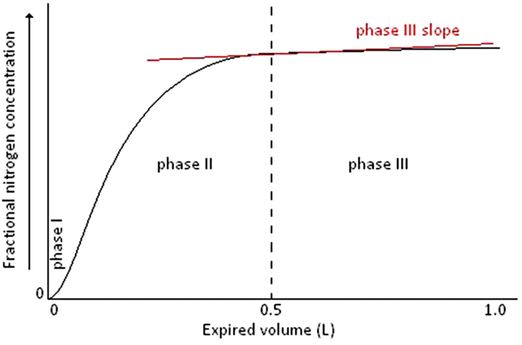
A single breath washout curve from the MBW test illustrating phase I, phase II, phase III, and the alveolar phase III slope (red)
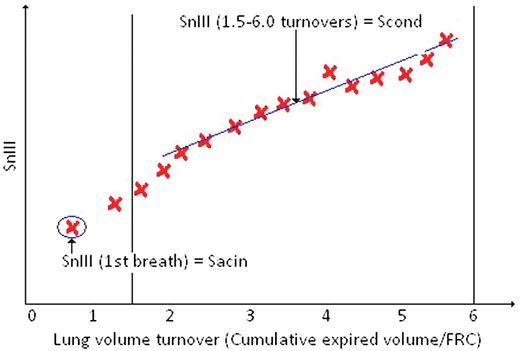
Phase III slope analysis illustrating the calculation of Scond and Sacin.
Phase I is the apparatus and airway dead space, phase II is the transition, and phase III is known as the alveolar plateau and equates to approximately 65 to 95% of the tidal volume of an expired breath. The phase III slope is calculated by regression over this region of the expired breath and is the change in gas concentration over that tidal volume. To compare these slopes over the entire MBW is not possible as the expired gas concentration of each breath decreases as the MBW progresses; therefore the phase III slope is normalized for gas concentration; this is known as the normalized phase III slope or SnIII. The SnIII values for each breath of the MBW test can then be plotted against the lung volume turnover (cumulative expired volume/FRC; Figure 14–8). Scond- and Sacin are derived from analysis of concentration SnIII of a multiple breath inert gas washout. The original work by Paiva and Engel provides in-depth description of this theory (9,10). In subjects with marked inhomogeneity occurring as a result of convection-dependent mechanisms the SnIII will increase steadily during the washout, as may be seen in cystic fibrosis (4). This is the result of uneven ventilation among the conducting airways.
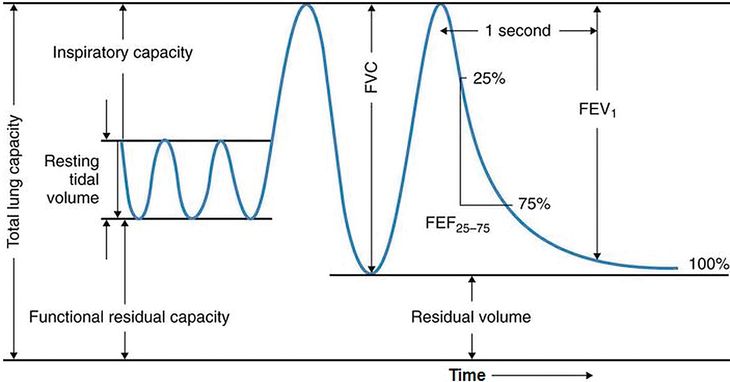
Spirogram showing volume on the vertical axis and time on the horizontal axis.
Thompson et al. speculated that the Scond value might be the result of the remodeling process that occurred in chronic asthma that did not respond to treatment, and the functional reflection of airway inflammation, within asthmatic airways (11). Further research is required before such speculative interpretations regarding structure–function relationships in the peripheral airways and acinus can be confirmed.
The ability to measure small airway function is important in a number of disease processes, for example, cystic fibrosis, asthma, and the lungs of preterm infants, all of which may result in changes within the small airways or acinar air spaces affecting airflow or ventilation efficiency within the airways. The small airway function variables measured in spirometry, such as FEF25–75% or forced expiratory flow at 50% of FVC (FEF50%), may be reduced in the presence of small airways disease, but there is mounting evidence that they are not sensitive or specific to disease processes occurring in small airways or airspaces, especially in the presence of airflow obstruction in the larger conducting airways or gas trapping within the lungs (11–16).
A standardization document published jointly by the ERS and ATS provides guidelines for washout equipment specifications, test performance, and analysis for both infant and adult populations and outlines the important theoretical background and principles essential for understanding gas washout techniques (17).
Lung Diffusing Capacity
Gas exchange is the primary function of the lung, and many lung diseases impair gas exchange. Respiration occurs externally between alveolar gas and pulmonary capillary blood and internally between systemic capillary blood and tissue. Measurements of arterial blood gas reflect the balance between internal and external respiration (6). Ventilation comprises not only gas involved in gas exchange (alveolar ventilation) but also gas that does not take part in gas exchange (dead-space ventilation). The balance between alveolar ventilation and carbon dioxide (CO2) production is shown in the arterial partial pressure of CO2 (PCO2) (6). The arterial partial pressure of oxygen (PO2) shows the adequacy with which the pulmonary blood flow is oxygenated by the lungs (6).
Diffusion is limited by the surface area over which diffusion occurs, capillary blood volume, hemoglobin concentration, and the properties of the lung parenchyma, for example, alveolar–capillary membrane thickness and/or the presence of excess fluid in the alveoli (6). The total lung volume is not involved in gas exchange. Most gas exchange occurs as a function of diffusion, not bulk flow. The role of ventilation is to provide bulk flow of gas with the ambient air and to provide a constant gradient for oxygen and carbon dioxide (6,18,19). Spirometry measures various parts of bulk flow, whereas diffusing capacity measures the forces at work in molecular movement with the oxygen concentration gradient from the alveolar surface through to the hemoglobin molecule. The test, diffusing capacity of the lung, commonly uses carbon monoxide as the tracer gas for measurement because carbon monoxide has a high affinity for binding to the hemoglobin molecule (6). This allows a measurement of pure diffusion because the movement of the carbon monoxide only depends on the properties of the diffusion barrier and the amount of hemoglobin (6).
Diffusing capacity of the lung for carbon monoxide (DLCO) is the measure of carbon monoxide transfer (19). In Europe, it is frequently called the transfer factor of carbon monoxide (TLCO). The commonly used clinical tests to measure DLCO are based on a ratio between the uptake of carbon monoxide in milliliters per minute divided by the average alveolar pressure of carbon monoxide at standard temperature and pressure, dry, per minute (STDP) (18).
Measurement of alveolar volume (VA) and pulmonary DLCO can provide a functional assessment of the volume and surface area available for gas exchange, which indirectly estimates the alveolar number and size (20). In subjects from around late school age to adulthood, DLCO and VA increase with somatic growth, such as height. These physiologic results are consistent with morphometric data that parenchymal lung growth occurs in this age range primarily by the increasing size of the existing alveoli (20,21). Factors that influence the diffusing capacity of the lung include increased ventilation–perfusion mismatch, reduction of alveolar surface area for gas exchange, decreased density of pulmonary capillaries, and a reduction in lung capillary blood flow (22). Interstitial lung diseases may result in abnormal DLCO long before spirometry or lung volume abnormalities are evident. Reduced DLCO is not only an abnormality of restrictive interstitial lung disease but also can occur in emphysema. Therefore other obstructive processes that mostly affect the airways can have similar spirometry, but a reduced DLCO implies a loss of alveolar surface area consistent with emphysema.
The most commonly used and standardized technique to measure DLCO is the single-breath breath-holding technique (18). In this test, a subject breathes in a known volume of tracer gas that typically contains 10% helium, 0.3% carbon monoxide, 21% oxygen, and the balance nitrogen (18). The subject breathes in the tracer gas and holds the breath for 10 seconds. The subject then exhales to wash out the mechanical and anatomic dead space, after which an alveolar sample is collected. DLCO is calculated from the total volume of the lung, breath-hold time, and the initial and final alveolar concentrations of carbon monoxide. The exhaled helium concentration is used to estimate a single-breath estimate of total lung capacity and the initial alveolar concentration of carbon monoxide (6,18). The driving pressure is assumed to be the calculated initial alveolar pressure of carbon monoxide (6). The calculated DLCO is a product of the patient’s single-breath estimate of total lung capacity multiplied by the rate of carbon monoxide uptake during the 10-second breath hold (6). This timed breath hold presents problems when attempting the measurement in infants, preschool children, and younger school-age children. A standardization document discussing single-breath determination of carbon monoxide uptake in the lung was published in 2005 as a result of a joint task force of the ERS/ATS and details recommendations relating to equipment requirements, study procedures, and reporting of data for plethysmographic measurements in infants (18).
Techniques for the Measurement of Lung Function in Preschool and School-Age Children
The level of cooperation required for many standard lung function tests means their application within the preschool group is difficult. Recent advances and development of commercially available equipment have led to an increase in the number of centers testing this age group. This will in turn lead to an increased knowledge of the practicalities involved in testing this age group.
Pulmonary function testing plays a key role in the diagnosis and management of chronic pulmonary conditions, such as asthma and cystic fibrosis, in children over 6 years of age.
However, objective physiologic assessments have a limited role in the care of infants and children under 6 years of age, due to the challenges of measuring lung function in these young patients. A number of lung function techniques have been developed and evaluated among children less than 6 years of age in the research setting and show promise as safe, feasible, and potentially useful clinical tests.
Preschool children present a number of special challenges. The children are generally too old to sedate for pulmonary function testing (PFT), as is done with infants, and measurement of lung function under anesthesia is neither ethically acceptable nor physiologically relevant to clinical management. Children in this age group are not able to voluntarily perform many of the physiological maneuvers required for the pulmonary function tests used in older children and adults. They have a short attention span and are easily distracted. Due to these issues, the children need to be engaged and encouraged by the operator to participate in the test.
Spirometry
Spirometry is commonly used to assess lung function in older children and adults, and there are several reasons why it is an appealing technique to apply to the preschool population. Most patients, especially those age 6 years and older, can easily perform spirometry when coached by an appropriately trained technician. The indications for spirometry are diverse; it can be used for diagnosing and monitoring respiratory symptoms and disease, for preoperative risk stratification, and as a tool in epidemiologic and other research studies. Spirometry is a voluntary maneuver in which a seated patient inhales maximally from tidal breathing to total lung capacity (TLC), and then rapidly exhales to the fullest extent until no further volume is exhaled at residual volume (RV) (Figure 14-8). The maneuver may be performed in a forceful manner to generate FVC.
Preschool children can successfully perform spirometry to identify disease states and track lung function over time. As with other lung function testing, equipment and testing conditions must be appropriate, and coaching must be provided by a skilled and experienced respiratory physiologist. Loeb et al. showed that the percentage of acceptable and repeatable spirometry increased with age, rising above 50% by age six, and reached a plateau with approximately 85% success at age ten (23). They also showed the most common unmet criteria for an unacceptable study among preschool children was glottic closure and nonmaximal efforts, while in school-age children it was failure to plateau (23). Recent advances in computer technology have allowed the introduction of visual incentive and interactive computer animation to aid the achievement of reliable and reproducible results in the preschool age group.
A spirogram is a graphic representation of air movement shown as a volume-time tracing or as a flow-volume tracing (Figure 14-9). Values produced in a spirogram provide important graphic and numeric data about the mechanical properties of the lungs, including airflow (FEV1, forced expiratory volume in 1 second) and exhaled lung volume. The measurement is normally expressed in liters for volumes or in liters per second for flows and is corrected for body temperature and pressure (BPTS) of gas that is saturated with water vapor. Data from a spirogram show important patterns that help distinguish obstructive pulmonary disorders that typically reduce airflow, such as asthma, from restrictive disorders that typically reduce total lung volumes, for example pulmonary fibrosis.
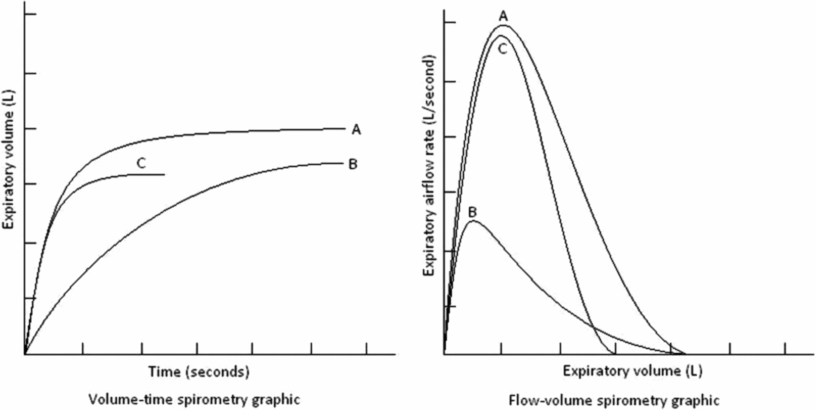
Spirometry patterns in various pulmonary disorders compared with normal, displayed as volume-time and flow-volume graphics. A – represents a normal pattern; B – represents an obstructive pattern; C – could either represent the pattern seen from preschool lung function or a restrictive disorder.
Abnormal spirograms are usually categorized as restrictive or obstructive impairments. An obstructive component implies airway obstruction, characterized by reduced expiratory flow rates. A restrictive pattern suggests a condition in which vital capacity (volume) is diminished. This must be distinguished from obstructive disease, diagnosed by measurement of normal or increased total lung capacity and decreased flow rates. The variables measured in spirometry are usually used to identify an obstructive pattern; the FEV1 is the most studied because it is easy to measure, is reproducible, and is sufficiently sensitive (23–26). This measurement is reduced in obstructive and restrictive disorders. In obstructive diseases, FEV1 is decreased disproportionately to the FVC, reducing the FEV1/FVC ratio indicating airflow limitation. In restrictive disorders, the FEV1, FVC, and total lung capacity are all decreased, and the FEV1/FVC ratio is normal or even high (27,28). FVC is a measure of lung volume and is typically reduced in diseases that cause the lungs to be smaller or reduce the amount of air a subject can inhale. These processes are generally termed restrictive and can include disorders of the lung parenchyma, such as pulmonary fibrosis, or of the ability to inhale, for example muscular weakness.
However, a reduced FVC is not always due to reduced total volumes and can be due to severe airflow obstruction and air trapping in large hyperinflated lungs, as in emphysema. When this occurs, the FVC is reduced because of reduced airflow, air trapping, and increased residual volume. Reduced FVC can occur despite a normal or increased total lung volume. Therefore, FVC is not a reliable indicator of total lung capacity or restriction, especially in the setting of airflow obstruction.
The shape of the flow-volume curve can indicate the location of airflow limitation, for example, the large upper airways or smaller airways (Figure 14-9). With common obstructive airflow disorders, such as asthma, the disease usually affects the expiratory loop and can reduce the effort-dependent peak expiratory flow as well as subsequent airflows that are independent of effort. The expiratory loop is typically concave in this instance. In contrast, several anatomic disorders that narrow the large airways can produce a variety of patterns of shortening or flattening of either the expiratory or inspiratory loop of the curve (variable upper airway obstruction) or both loops of the curve (fixed upper airway obstruction). Other measurements reflect small airways, such as measures of flow from a spirogram, like the maximal midexpiratory flow (MMEF) or forced expiratory flow at 25% to 75% vital capacity (FEF25–75%). The FEF25–75% is the slope of the spirogram when between 25% and 75% of the FVC has been expired. The FEF25–75% is a more sensitive early indicator of airway obstruction than FEV1, but it is less reproducible (25). The ATS/ERS have produced guidelines that outline acceptability and reproducibility criteria, although based mostly on spirometry when performed in adults (25) and a modification specific to spirometry measured in preschool children (29).
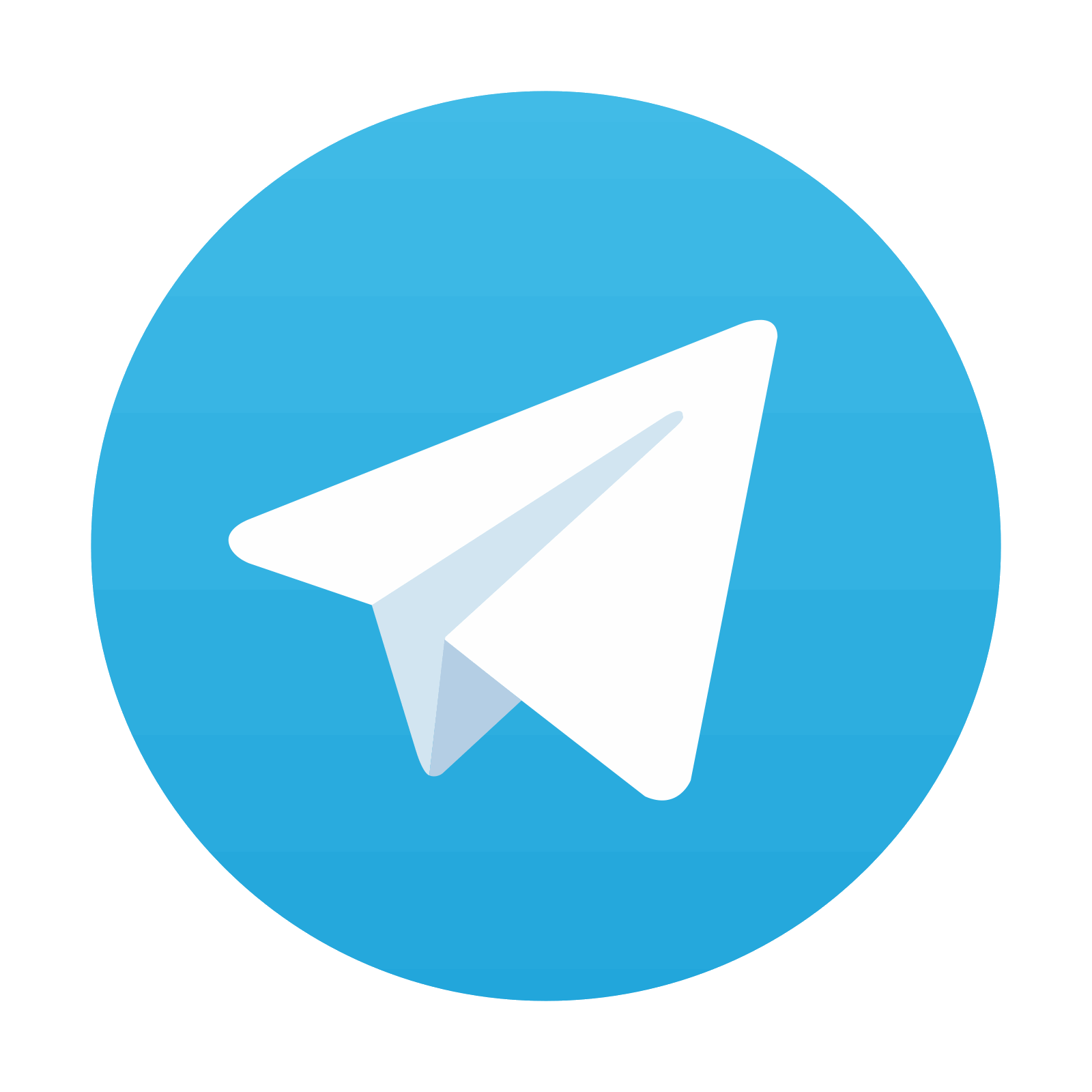
Stay updated, free articles. Join our Telegram channel
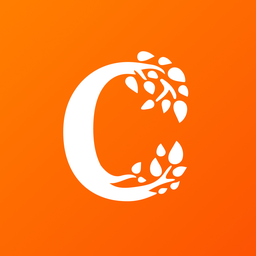
Full access? Get Clinical Tree
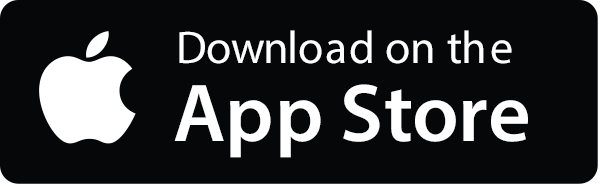
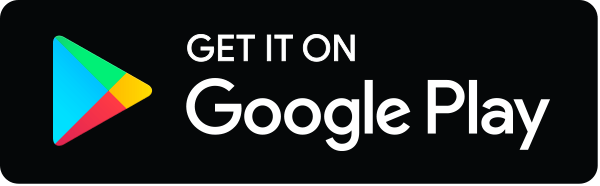