Fig. 15.1
A cartoon illustrating roughly how humans regulate temperature. Temperature is sensed at the skin surface, deep tissues, the spinal cord, the brain, and the hypothalamus. Integration of thermal input occurs at various levels, but the hypothalamus is the most important controller in mammals. The most important efferent autonomic responses are sweating, arteriovenous shunt vasoconstriction, and shivering. Behavioral responses (volitional activity) are by far the strongest defenses but are not usually available to surgical patients. Each response is characterized by its threshold (triggering core temperature), gain (increase in response intensity with further deviation in core temperature), and maximum response intensity (Reprinted with permission, Cleveland Clinic Center for Medical Art & Photography ©2017. All Rights Reserved)
Afferent Input
Temperatures are sensed peripherally and throughout the body by various receptors and nerves, with transient receptor potential (TRP) proteins being the most important. The TRPV1 receptor was identified in 1997. Since then, ten TRP channels (TRPV1, TRPV2, TRPV3, TRPV4, TRPM2, TRPM3, TRPM4, TRPM5, TRPM8, and TRPA1) have been reported to be highly temperature sensitive. Five of them—TRPV1, TRPV2, TRPM3, TRPM8, and TRPA1—are expressed on human sensory neurons. Some thermo-TRP channels are probably either the prime or sole molecular thermosensors responsible for the reception of peripheral temperature [12–14].
Warm afferent signals are conveyed by unmyelinated C-fibers, as is pain. In contrast, cold signals traverse myelinated A-delta fibers; both C-fibers and A-delta fibers are widely distributed [15]. Most thermal input is conducted along the spinothalamic tracts, although both afferent and efferent thermal signals are diffusely distributed within the neuraxis [16].
The central thermoregulatory control system accepts thermal input from tissues all over the body. The relative contributions of most tissues have yet to be determined in humans. However, animal studies suggest that the hypothalamus, other portions of the brain, the spinal cord, and deep thoracic and abdominal tissues each contribute very roughly 20% [8, 17–19].
The mean skin temperature contributes 5–20% as much as core temperature (deep central tissues and brain) to control sweating and active vasodilation; furthermore, the relation between mean skin and core temperatures at response thresholds is linear [4, 20–23]. That is, a 1 °C increase in skin temperature reduces the sweating and active capillary vasodilation thresholds (expressed in terms of core temperature) by 0.05–0.2 °C. Arithmetically, this relation takes the form
where ThresMBT is the sweating or vasodilation threshold in terms of physiologic (rather than anatomic) mean body temperature, T skin is the mean skin temperature, and T core is the core temperature, all in degrees centigrade.

The proportionality constant, β, in this case is 0.05–0.2. The skin surface contributes 20% ± 6% to control of vasoconstriction and 19% ± 8% to control of shivering; the contribution in linear (Fig. 15.2) [19]. Regional sensory contributions to thermoregulatory control have not been specifically evaluated in the elderly. However, there is little reason to believe that temperature sensation fails in the elderly or that integration differs markedly.


Fig. 15.2
The relative contribution of mean skin temperature to control thermoregulatory vasoconstriction and shivering in six men. The threshold (triggering core temperature) for each response is plotted vertically against mean skin temperature. Core and skin temperatures at the vasoconstriction and shivering thresholds were linearly related. The extent to which mean skin temperature contributed to central thermoregulatory control (β) was calculated from the slopes (S) of the skin temperature versus core temperature regressions, using the formula: β = S/(S − 1). Cutaneous contribution to vasoconstriction averaged 20% ± 6%, which did not differ significantly from the contribution to shivering: 19% ± 8% (Reprinted from Cheng et al. [19]. With permission from Wolters Kluwer Health, Inc.)
Central Control
Thermal afferent signals are integrated at numerous levels within the neuraxis, including the spinal cord and brain stem. The dominant controller in mammals, however, is the hypothalamus. (Interestingly, the spinal cord dominates in birds.) Although core temperature varies with a daily circadian rhythm [24], body temperature is normally controlled to within a few tenths of a degree centigrade almost irrespective of the environment [22]. Such precise control is maintained by a powerful thermoregulatory system incorporating afferent inputs, central control, and efferent defenses.
The thresholds triggering thermoregulatory defenses are uniformly about 0.3 °C greater during the follicular phase in women [25], who then have core temperatures an additional ≈1 °C greater than men during the luteal phase [26]. However, men and women regulate core body temperature with comparable precision, usually maintaining core temperature within a few tenths of a °C of the target temperature (Fig. 15.3).


Fig. 15.3
The thresholds (triggering core temperatures) for the three major autonomic thermoregulatory defenses: sweating , vasoconstriction , and shivering . Temperatures between the sweating and vasoconstriction threshold define the interthreshold range, temperatures not triggering autonomic responses. The thresholds are uniformly about 0.3 °C greater during the follicular phase in women than in men and would be an additional ≈0.5 °C greater during the luteal phase. However, men and women regulate core body temperature with comparable precision. Results are presented as means ± SD (Reprinted from Lopez et al. [25]. With permission from Wolters Kluwer Health, Inc.)
The major autonomic warm defenses, sweating and active vasodilation, are triggered at about the same temperature and seem to operate synchronously [27]. In contrast, vasoconstriction is the first autonomic response to cold [25]. Only when vasoconstriction is insufficient to maintain core temperature in a given environment is nonshivering thermogenesis or shivering initiated. In humans, nonshivering thermogenesis is restricted to infancy; infants use this defense in preference to shivering [28]. In contrast, nonshivering thermogenesis is of little importance in adult humans [29–31], although it is the most important cold defense in small animals.
When one efferent response is inadequate to maintain core temperature in a given environment, others are activated. Similarly, secondary defenses compensate for those working poorly. For example, when arteriovenous shunt vasoconstriction is defeated by administration of a vasodilating drug, core hypothermia will initiate shivering. Because autonomic responses are to some extent compromised in the elderly, behavioral responses are probably more important in this population—although this theory has yet to be formally evaluated.
Efferent Responses
Sweating is mediated by postganglionic cholinergic nerves that terminate on sweat follicles [32]. These follicles apparently have no purpose other than thermoregulation. In this regard, they differ from most other thermoregulatory effectors which are co-opted by the thermoregulatory system but continue to have other important roles, for example, vasomotion in blood pressure control or skeletal muscles in postural maintenance.
Heat exposure can increase cutaneous water loss from trivial amounts to 500 mL/h. Losses in trained athletes can even exceed 1 L/h. In a dry, convective environment, sweating can dissipate enormous amounts of heat—perhaps up to ten times the basal metabolic rate. Sweating is the only thermoregulatory defense that continues to dissipate heat when environmental temperature exceeds core temperature.
Active precapillary vasodilation is mediated by a factor, probably nitric oxide [33, 34], released from sweat glands, and thus occurs synchronously with sweating. Active dilation can increase cutaneous capillary flow enormously, perhaps to as much as 7.5 L/min [35]. The purpose of this dilation, presumably, is to transport heat from muscles and the core to the skin surface where it can be dissipated to the environment by evaporation of sweat.
Active arteriovenous shunt vasoconstriction is adrenergically mediated. The shunts are 100-μm-diameter vessels that convey 10,000 times as much blood as a comparable length of 10-μm capillary (laminar flow increases by the fourth power of vessel radius) [5]. Anatomically, they are restricted to the fingers, toes, nose, and nipples. Despite this restriction, shunt vasoconstriction is among the most frequently used and important thermoregulatory defenses. The reason is that the blood traversing shunts in the extremities must flow through the arms and legs, thus altering the heat content of these large tissue masses.
Shivering is an involuntary, thermogenic tonic tremor [7]. Typically, it doubles metabolic rate [36, 37], although greater increases can be sustained briefly. The shivering threshold is normally ≈1 °C less than the vasoconstriction threshold, suggesting that it is activated only under critical conditions and is not the preferred means of maintaining core temperature. One reason may be that shivering is a relatively inefficient response. Although shivering effectively transfers metabolic energy into heat , the heat is largely produced in the periphery where the largest muscles are located. Loss of the peripherally produced heat to a cold environment is further accentuated by the metabolic needs of shivering muscle and the resulting vasodilation.
Impaired Thermoregulation in the Elderly
There is considerable epidemiologic evidence that the elderly often fail to adequately regulate body temperature. Accidental hypothermia is especially likely in three populations: drug abusers (especially alcoholics), people suffering from extreme exposure (such as cold-water immersion), and the elderly [38]. While extreme—and usually prolonged—cold exposure is required to produce clinical hypothermia in young, healthy individuals, serious hypothermia is common among alcohol abusers even with mild exposure [39]. Hypothermia in these patients presumably results from drug-induced inhibition of thermoregulatory defenses. The extent to which alcohol impairs autonomic defenses remains controversial [40–43]; however, at minimum, alcohol significantly impairs appropriate behavioral responses to cold exposure.
Hypothermia in the elderly can occur in moderately cold environments and is typically not associated with drug use [38, 39]. This observation suggests that hypothermia in the elderly may result from age-induced thermoregulatory failure. Supporting this thesis is the work of MacMillan et al. [44] who demonstrated in 1967 that elderly victims of accidental hypothermia responded abnormally to cold challenge. Subsequent studies have demonstrated that cold exposure produces more hypothermia in the elderly than in younger subjects [45, 46]. Cold tolerance is also poor in elderly rats [47].
Excessive hypothermia in the elderly presumably results from inadequate activation or efficacy of thermoregulatory defenses. Consistent with this theory, several features of thermoregulatory control in the elderly are known to differ from those in younger subjects. Sweating thresholds remain normal to the age of ≈70 years; however, the sweating rate is reduced in the elderly. Age-relative reduction in the sweating rate seems to depend on fitness level [48]—although fitness level may itself depend on overall health. Decreased gain results from reduced sweat production per activated gland, rather than recruitment of fewer glands [49]. Sweating is also less effective in children than in adolescents [50]. Other studies, however, failed to identify age-related differences in sweating [51].
Vasoconstriction in response to cold exposure is reduced in the elderly [45]. This is a clinically important observation because vasoconstriction is the primary autonomic response to cold exposure. Similarly, the shivering threshold is reduced in the elderly [52]. Interestingly, abnormally reduced thresholds were not apparent in subjects younger than 80 years of age, and even then they were apparent in only a fraction of the population (Fig. 15.4). These data suggest that age-related thermoregulatory impairment may not be common at ages less than 80 years. The data further suggest that impairment is not a linear function of age but instead occurs unpredictably in a fraction of the elderly population.


Fig. 15.4
The effect of aging on the shivering threshold. Fifteen patients aged <80 years (58 ± 10 years) (mean ± SD) shivered at 36.1 ± 0.6 °C; in contrast, ten patients aged ≥80 years (89 ± 7 years) shivered at a significantly lower mean temperature, 35.2 ± 0.8 °C (p < 0.001). The shivering thresholds in 7 of the 10 patients aged more than 80 years was <35.5 °C, whereas the threshold equaled or exceeded this value in all the younger patients (Reprinted from Vassilieff et al. [52]. With permission from Wolters Kluwer Health, Inc.)
Altogether, there are surprisingly few studies evaluating age-related thermoregulatory changes in humans, especially in subjects exceeding 80 years of age. Even fewer of the studies are recent and use modern methods of controlling (or compensating) for changes in skin temperature. Most do not distinguish altered thresholds from reduced gain or maximum response intensity. The ethical and practical difficulties of conducting controlled physiologic evaluations in the elderly are apparent, and these difficulties are magnified in extremely old subjects who are most likely to have impaired responses. Nonetheless, as a large fraction of the US population enters this age bracket, greater understanding of age-dependent thermoregulatory inhibition is clearly required.
Thermoregulation During Anesthesia
Thermoregulatory Defenses During Anesthesia
General anesthetics and most sedatives slightly increase the threshold for warm-defense responses. But these drugs also markedly decrease cold-response thresholds, thus increasing the interthreshold range 10–20-fold to ≈4 °C at typical doses of common anesthetics. Because temperatures within this range do not trigger autonomic thermoregulatory defenses (by definition) and because behavioral compensations are unavailable in anesthetized patients, body temperature perturbations are common during anesthesia.
The first thermal problem identified with surgery was hyperthermia [53]. Hyperthermia resulted in part from the frequent use of ether, a drug associated with substantial sympathetic nervous system activation and thus peripheral vasoconstriction. More importantly, however, hyperthermia resulted when anesthetic-induced thermoregulatory impairment was combined with a warm operating environment. This mechanism continues to produce clinically important hyperthermia in some developing countries, although ether has largely been supplanted by halothane. Hyperthermia in developed countries gave way to hypothermia, however, with the introduction of air conditioning. Hypothermia is now by far the most common perioperative thermal disturbance and results from anesthetic-induced inhibition of thermoregulatory defenses combined with a cold surgical environment.
Sedatives and general anesthetics, with the exception of midazolam [54], markedly impair thermoregulatory control. For example, the sweating threshold is linearly increased by propofol [55], alfentanil [56], isoflurane [27], and desflurane [57]. Reduction of the vasoconstriction and shivering thresholds is also a linear function of propofol [55], dexmedetomidine [58], meperidine [59], and alfentanil [56] concentrations. Desflurane and isoflurane, however, produce a nonlinear reduction in the major cold-response thresholds, reducing the vasoconstriction and shivering thresholds disproportionately at higher anesthetic concentrations (Fig. 15.5) [57]. The result is that clinical doses of all anesthetics and most any “balanced” combination of anesthetics and opioids markedly increase the interthreshold range—thus substantially impairing thermoregulatory defenses.


Fig. 15.5
Thermoregulatory response thresholds during desflurane anesthesia. The sweating threshold increased linearly, but slightly, during desflurane anesthesia. Desflurane markedly—although nonlinearly—reduced the vasoconstriction threshold. Consequently, the interthreshold range (temperatures not triggering autonomic thermoregulatory defenses) increased enormously during desflurane administration. In contrast, the vasoconstriction-to-shivering range remained essentially unchanged. Results are presented as means ± SD. MAC minimal anesthetic concentration (Reprinted from Annadata et al. [57]. With permission from Wolters Kluwer Health, Inc.)
Anesthetic-Induced Thermoregulatory Impairment in the Elderly
Intraoperative hypothermia is more common and severe in the elderly [60]. Because a major cause of intraoperative hypothermia is anesthetic-induced inhibition of thermoregulatory responses, these two observations suggest that anesthetics impair thermoregulation more in the elderly than in young patients. This thesis is supported by the observation that the vasoconstriction threshold is approximately 1 °C lower in elderly surgical patients than in younger ones (Fig. 15.6) [61].


Fig. 15.6
The effect of aging on thermoregulatory vasoconstriction during general anesthesia. The vasoconstriction threshold was significantly less in the elderly (33.9 ± 0.6 °C, mean ± SD) than in younger patients (35.1 ± 0.3 °C) (p < 0.01). Filled squares indicate the vasoconstriction threshold in each patient; the open circles show the mean and standard deviations in each group (Reprinted from Kurz et al. [61]. With permission from Wolters Kluwer Health, Inc.)
Intraoperative hypothermia is not only more common in the elderly, but it lasts longer postoperatively [62]. It is also associated with less shivering when compared to younger patients [60, 63], and what shivering does occur is at a lower intensity [64]. Prolonged hypothermia without shivering suggests that thermoregulatory defenses are not being activated, which is consistent with reduced perioperative vasoconstriction [61] and shivering [52] thresholds in the elderly.
An additional factor to consider is the age-dependent effects of anesthetic drugs. Renal and hepatic function is often reduced in the elderly. Consequently, clinically important plasma concentrations are likely to persist longer and at higher levels in the elderly. Equally important, any given plasma concentrations of many drugs produce a greater effect in the elderly. The minimum alveolar concentration of volatile anesthetics, for example, decreases about 25% in the elderly [65, 66]. Similarly, the effect of midazolam is markedly age dependent [67]. Combined pharmacokinetic and pharmacodynamic augmentation of anesthetic drug effects is thus likely to further impair thermoregulation in the elderly.
Perioperative Heat Balance
Both physical and physiologic factors contribute to perioperative hypothermia . Hypothermia would be unlikely without anesthetic-induced inhibition of thermoregulatory control because thermoregulatory defenses would normally be sufficient to prevent core temperature perturbations even in a cool operating room environment. However, most anesthetics markedly increase the range of temperatures not triggering thermoregulatory defenses [68]. Within this interthreshold range, body temperature changes are determined by patients’ physical interactions with their immediate environments. Larger operations and colder rooms are thus associated with greater hypothermia. Once triggered, however, thermoregulatory vasoconstriction usually prevents further hypothermia—no matter how large and long the operation might be [69].
Despite multiple modalities of heat loss, each described by different (and mostly nonlinear) equations, cutaneous heat loss in patients is a roughly linear function of the difference between skin and ambient temperatures. The physical laws and equations characterizing heat transfer are comparably valid for all animate and inanimate substances and, of course, apply equally in young and elderly patients.
Mechanisms of Heat Transfer
There are four types of heat transfer: radiation, convection, conduction, and evaporation [70]. Among these, radiation and convection are by far the most important during surgery, together accounting for approximately 85% of the total loss [71]. Fractional losses via each route are, however, determined by numerous physical and physiologic factors including incision size, amount of administered (cold) intravenous fluid, and thermoregulatory vasoconstriction.
Radiative losses are mediated by photons and do not depend on any intervening media. Losses via this mechanism are related to surface properties (emissivity) and the difference of the fourth power of exposed skin and wall temperatures (in degree Kelvin). Radiative losses are thus not directly influenced by ambient temperature, although ambient temperature indirectly influences both wall and skin temperature. Radiation probably contributes about 60% to total heat loss [71, 72].
Conduction is defined by direct transfer of heat energy between opposing surfaces. It is related only to the insulating properties of the surfaces (or of an intervening layer) and the temperature difference between the surfaces. It is unlikely that conduction contributes more than about 5% to overall heat loss in the perioperative period. The reason conduction contributes so little is that only a small fraction of the body surface area is in direct contact with another solid surface and that surface is likely to be the operating table mattress which is a good insulator. The body heat required to warm cold intravenous fluids is probably best considered as a conductive loss. Loss via this route usually exceeds conventional surface-to-surface heat transfer.
Convection, which is often termed “facilitated conduction,” contributes considerably more than conduction, perhaps about 25% of the total loss. Normally, there is essentially no conduction into air because still air is an excellent insulator and because a small layer of still air is maintained adjacent to the skin surface. But when warm air next to the skin is pushed away, it is replaced by cool air from the surrounding environment. This air is itself warmed by extracting heat from the skin, only in turn to be replaced by additional cool air. The equation describing convection is similar to that characterizing conduction, with the addition of a factor for the square root of air speed. Convection is the basis for the familiar “wind chill factor.”
The heat of vaporization of water is among the highest of any substance: 0.58 kcal/g. Evaporation of large amounts of water thus absorbs enormous amounts of heat, which is why sweating is such an effective defense against heat stress. But except in infants, insensible cutaneous water loss is negligible [73, 74], and evaporative heat loss constitutes only a tiny fraction of the total in non-sweating individuals. Evaporative loss contributes to surgical hypothermia during skin preparation when the skin surface is scrubbed with water- or alcohol-based solution that is subsequently allowed to evaporate. Because skin preparation is usually restricted to a relatively small area and because evaporation is permitted for only a brief time, heat loss from skin preparation is not usually clinically important [75].
Water is also vaporized and lost from the lungs when they are ventilated with dry, cold gases. Numerous clinical studies [76, 77] and thermodynamic calculations [78] indicate that respiratory heat loss in adults is less than 10% of the total heat loss. Other studies identify effects of airway heating and humidification on core temperature that seem difficult to reconcile with thermodynamic calculations of heat transfer [79–81]; in some cases, these aberrant results are attributable to study design flaws. In contrast, respiratory losses are somewhat more important in infants and children than in adults [82, 83].
Finally, heat is lost when water evaporates from exposed surfaces within surgical incisions. The extent of this loss in humans remains unknown, although clinical experience suggests that it may be substantial because patients undergoing large operations become considerably more hypothermic than those having smaller procedures. Evaporative loss from large incisions may be up to half of the total heat loss in animals [84], although this ratio is likely less in humans.
Distribution of Heat Within the Body
Intraoperative hypothermia develops with a characteristic three-phase pattern. The first is a rapid, 1–1.5 °C decrease in core temperature occurring during the first hour after induction of anesthesia [85]. This is followed by a slower, nearly linear decrease in core temperature lasting 2–3 h [86]. And finally, core temperature reaches a plateau and does not decrease further [69]. Each portion of this curve has a different etiology.
The initial, rapid decrease in core temperature after induction of general anesthesia results from core-to-peripheral redistribution of body heat. Redistribution results when anesthetic-induced inhibition of tonic thermoregulatory vasoconstriction allows heat to flow from the relatively warm core thermal compartment to cooler peripheral tissues. (Surprisingly, anesthetic-induced vasodilation increases cutaneous heat loss only slightly [87].) Although redistribution, by definition, does not alter body heat content, it does markedly decrease core temperature. Internal redistribution of body heat is a major cause of core hypothermia in most patients (Fig. 15.7) [85]. Redistribution is also a major cause of hypothermia during epidural anesthesia.


Fig. 15.7
Changes in body heat content and distribution of heat within the body during induction of general anesthesia. Heat loss and metabolic heat production were initially similar. Overall heat balance was thus near zero before induction of anesthesia (at elapsed time zero), but subsequently decreased by ≈31 kcal/h. The contributions of decreased overall heat balance and internal redistribution of body heat to the decrease in core temperature were separated by multiplying the change in overall heat balance by body weight and the specific heat of humans. The resulting change in mean body temperature (“mean body”) was subtracted from the change in core temperature (“core”), leaving the core hypothermia specifically resulting from redistribution (“redistribution”). After 1 h of anesthesia, core temperature had decreased by 1.6 ± 0.3 °C, with redistribution contributing 81% to the decrease. During the subsequent 2 h of anesthesia, core temperature decreased an additional 1.1 ± 0.3 °C, with redistribution contributing only to 43%. Redistribution thus contributed 65% to the entire 2.8 ± 0.5 °C decrease in core temperature during the 3 h of anesthesia. All results are shown as means ± SD (Reprinted from Matsukawa et al. [85]. With permission from Wolters Kluwer Health, Inc.)
The 2–3-h-long linear decrease in core temperature results simply from heat loss exceeding heat production [76]. In part, this results from an ≈30% reduction in metabolic heat production during general anesthesia [85]. Metabolic heat production is nearly constant during anesthesia and minimally influenced by anesthetic technique [69, 88]. Respiratory heat loss (even with a nonrebreathing circuit and unwarmed, dry gases) is simply a linear function of metabolic rate. In contrast, cutaneous heat loss is determined largely by surface insulation and ambient temperature and can therefore be altered by anesthetic management. The slope of this second phase of hypothermia curve depends on the difference between metabolic heat production and cutaneous and respiratory heat loss. While typically negative, the slope can be positive when heat loss is reduced to below metabolic heat production by high ambient temperature, sufficient insulation, or effective warming systems.
After 3–4 h of anesthesia, core temperature usually reaches a plateau and does not decrease further. This plateau is generally associated with arteriovenous shunt vasoconstriction. Vasoconstriction contributes to the plateau via two distinct mechanisms. The first is simply decreasing cutaneous heat loss [89]. The second is by constraining metabolic heat to the core thermal compartment, thus re-forming the normal core-to-peripheral temperature gradient that was obliterated by the initial redistribution hypothermia. Because heat loss may continue to exceed heat production during the core temperature plateau, body heat content often continues to decrease during this period—even though core temperature is constant (Fig. 15.8) [69]. For further discussion of perioperative heat balance, readers are referred to a detailed review [89].


Fig. 15.8
Changes in body heat content and distribution of heat within the body during the core temperature plateau in anesthetized subjects. Vasoconstriction decreased cutaneous heat loss by ≈25 kcal/h. However, heat loss exceeded heat production throughout the study. Consequently, mean body temperature, which decreased at a rate of ≈0.6 °C/h before vasoconstriction, subsequently decreased at a rate of ≈0.2 °C/h. Core temperature also decreased at a rate of ≈0.6 °C before vasoconstriction but remained virtually constant during the subsequent 3 h. Because mean body temperature and body heat content continued to decrease, constraint of metabolic heat to the core thermal compartment contributed to the core temperature plateau. That is, vasoconstriction reestablished the normal core-to-peripheral temperature gradient by preventing metabolic heat (which is largely generated in the core) from escaping to peripheral tissues. Constrained heat is presented cumulatively, referenced to the onset of intense vasoconstriction defined as time zero; data are expressed as means ± SD (Reprinted from Kurz et al. [69]. With permission from Wolters Kluwer Health, Inc.)
Benefits of Mild Hypothermia
Severe hypothermia (i.e., core temperatures near 28 °C) has been known for decades to be protective against cerebral ischemia [90]. The basis for this protection was thought to be a decrease in the cerebral metabolic rate to about half of normal levels [91]. Although decreased metabolic rate surely contributes to hypothermic protection, there is increasing evidence that other mechanisms contribute. These include decreased release of excitatory amino acids (such as glutamate) and free fatty acids [92, 93], inhibition of calcium-/calmodulin-dependent protein kinase II [94], preservation of the blood–brain barrier, [95, 96] reduced synthesis of nitric oxide [97] and ubiquitin [98].
More than 100 animal studies in virtually every ischemic model demonstrate that just 1–3 °C brain hypothermia provides substantial protection against ischemia [93, 99–102]. In each case, the protection seems to far exceed that resulting simply from reduced metabolic rate. There is also evidence that mild hypothermia protects the spinal cord and liver against ischemia [103]. Furthermore, mild hypothermia seems protective during spinal cord ischemia [104] and is beneficial during hypoxia and shock.
The extent to which mild hypothermia ameliorates cerebral ischemia in humans remains unclear. The best documented indication is neonatal asphyxia [105–108]. Two major trials suggested that mild hypothermia improves neurological function after cardiac arrest [109, 110]. However, a subsequent much larger trial showed no benefit whatsoever [111]. Trials have failed to show benefit from therapeutic hypothermia for aneurism surgery [112], brain trauma [113], and acute myocardial infarction [114]. All these trials, though, had substantial limitations, and negative outcomes in the studied contexts do not necessarily mean that the general concept of hypothermic brain protection is flawed.
Hypothermia clearly reduces intracranial pressure, but a randomized trial of hypothermia in patients with critically elevated intracranial pressure did not improve long-term outcome [115]. Major trials on mild hypothermia are in progress for stroke, sepsis, and various other conditions. Assuming benefit is eventually demonstrated, it seems likely that the elderly will be at greater risk of ischemia because of age-related vascular compromise while simultaneously being at greater risk of hypothermia-related complications. In the absence of specific data, clinicians may have to make difficult risk–benefit judgments in elderly patients.
Complications of Hypothermia
Coagulopathy and Allogeneic Transfusion Requirement
Hypothermia decreases platelet function [116], apparently by decreasing the release of thromboxane A2 [117]. This effect on platelet function seems to be entirely related to local, rather than core, temperature. (This is a factor that should be considered when interpreting a bleeding time.) Hypothermia also directly inhibits the enzymes of the coagulation cascade [118, 119] The effects of hypothermia on bleeding have generally not been appreciated by clinicians, in large part because coagulation tests are performed at 37 °C, irrespective of patients’ actual temperatures.
Hip arthroplasty is among the most common operations in the elderly, and it is a procedure associated with substantial blood loss. Just 2 °C core hypothermia substantially increases perioperative blood loss during total hip arthroplasty, and also increases the requirement for allogeneic blood transfusion, as shown in some [120, 121] but not all [122] studies (Table 15.1). A meta-analysis summarizes available trial results [123], and a large observational study indicates that transfusion requirements increase with hypothermia [124].
Table 15.1
Mild intraoperative hypothermia increases blood loss during hip arthroplasty
Normothermic | Hypothermic | p value | |
---|---|---|---|
Final intraoperative core temperature (°C) | 36.6 ± 0.4 | 35.0 ± 0.5 | <0.001 |
Blood loss (L) | 1.7 ± 0.4 | 2.2 ± 0.6 | <0.001 |
Allogeneic blood (mL/patient) | 10 ± 55 | 80 ± 154 | <0.02 |
Surgical Wound Infections and Duration of Hospitalization
Wound infection is a common and serious complication of anesthesia and surgery. In patients having colon surgery, the risk of wound infection ranges from 3 to 22% [125]. Infections typically prolong hospitalization by 5–20 days per infection and substantially increase cost [126, 127]. Hypothermia facilitates perioperative wound infections in two ways. First, sufficient intraoperative hypothermia triggers thermoregulatory vasoconstriction [128] and postoperative vasoconstriction is universal in hypothermic patients [129]. Vasoconstriction decreases tissue oxygen partial pressure which reduces resistance to infection [130, 131]. Second, mild core hypothermia directly impairs numerous immune functions [132, 133].
Finally, vasoconstriction-induced tissue hypoxia also decreases wound strength independently of its effect on resistance to infection. Scar formation requires proline and lysine hydroxylation, permitting the cross-linking between collagen strands that provides wound tensile strength [134]. The hydroxylases catalyzing this reaction are oxygen tension dependent [135]. Collagen deposition is thus proportional to arterial PO2 in animals [136] and to wound tissue oxygen tension in humans [137].
Consistent with these in vitro data, mild hypothermia during anesthesia reduces resistance to Escherichia coli and Staphylococcus aureus inoculations in guinea pigs [138, 139]. Furthermore, just 2 °C core hypothermia tripled the incidence of wound infection in patients having colon surgery (Table 15.2) [140]. The adverse effect of hypothermia on infection, especially non-wound infections, is supported by a recent retrospective analysis of compliance with thermal management guidelines. Compliant patients proved to have a significantly lower risk of hospital-acquired infection, shorter hospitalizations, and reduced mortality [141].
Table 15.2
Mild intraoperative hypothermia increases the incidence of surgical wound infections and the duration of hospitalization
Normothermic | Hypothermic | p value | |
---|---|---|---|
Final intraoperative core temperature (°C) | 36.6 ± 0.5 | 34.7 ± 0.6 | <0.001 |
Infections/number of patients | 6/105 | 18/95 | <0.01 |
Duration of hospitalization (days) | 11 ± 4 | 14 ± 4 | <0.01 |
Postoperative Shivering
It is common in reviews and book chapters to include the following logic: (1) shivering increases metabolic rate “up to 400%”; and (2) increased metabolic rate could be detrimental to elderly patients having cardiovascular disease [142, 143]. However, postoperative shivering in elderly patients is relatively rare [64] and usually of low intensity when it does occur. On average, postoperative shivering in young patients doubles oxygen consumption (although higher values may occasionally be sustained) [144, 145], whereas metabolic rate increases only ≈20% in the elderly [64]. Thus, there seems to be little support for the theory that elderly patients who become hypothermic subsequently develop shivering-induced myocardial ischemia.
Some patients, nonetheless, shiver during recovery from general anesthesia. At the very least, shivering is uncomfortable and remembered by many patients as one of the worst aspects of their surgical experience. Most postoperative shivering-like tremor is thermoregulatory [129], and therefore, can be completely prevented by maintaining intraoperative normothermia [146]. However, there is a small incidence of low-intensity tremor that is not thermoregulatory [147], a tremor that correlates with inadequate treatment of surgical pain [148]. A similar non-thermoregulatory shivering-like tremor can be observed during epidural analgesia for labor [149].
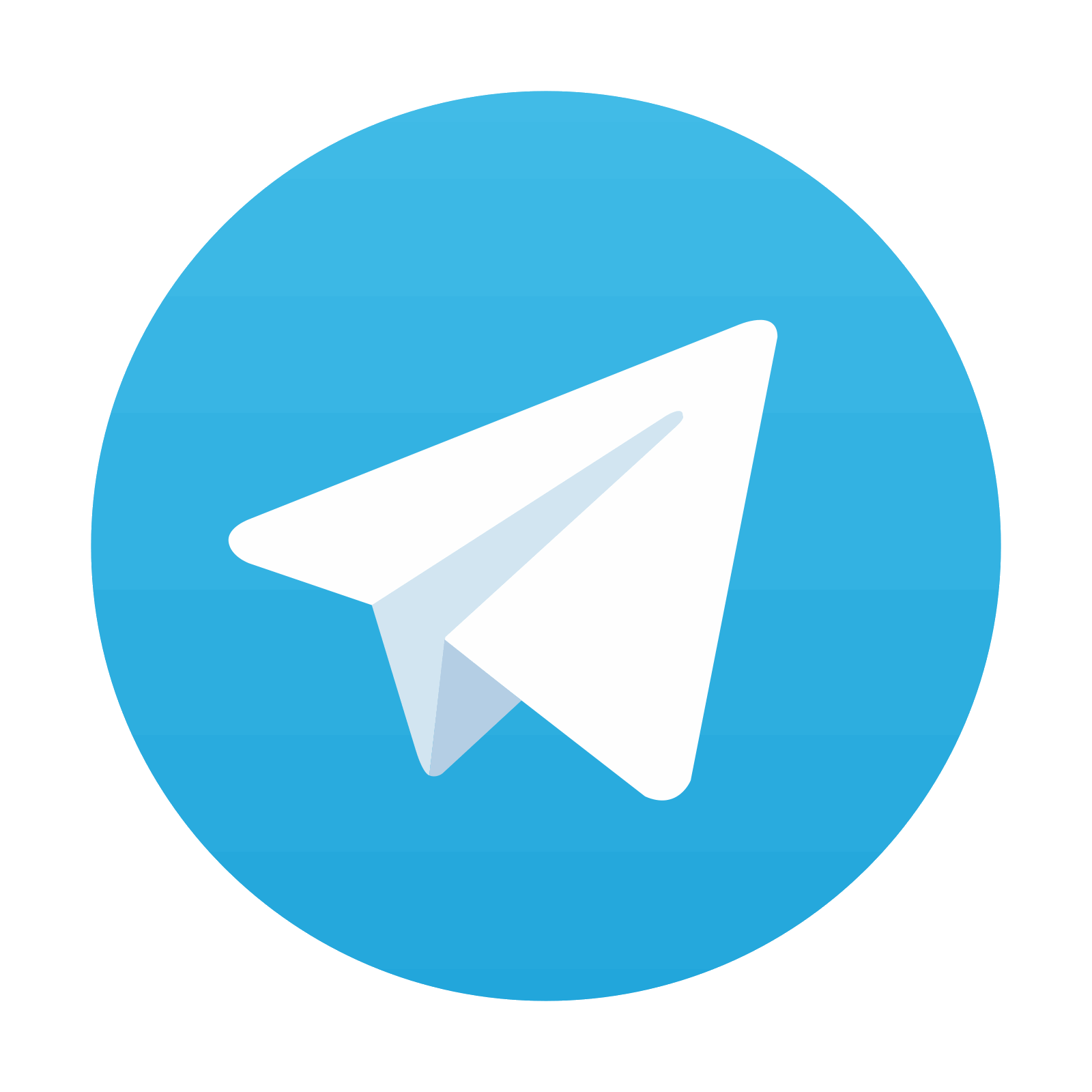
Stay updated, free articles. Join our Telegram channel
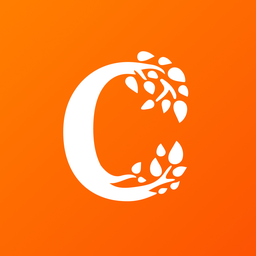
Full access? Get Clinical Tree
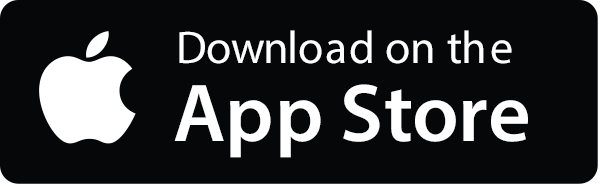
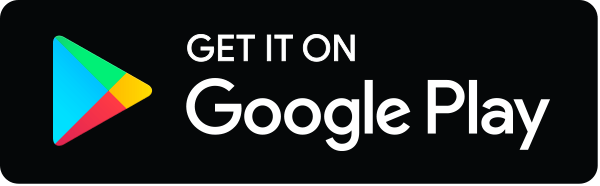