Keywords
Early embryo hemodynamics in chickZebrafishMouseHuman, vitelline artery pressureVentricular pressureCardiac outputTissue metabolic demandsPeripheral heartsPlacental circulationAcardiac twinTRAP (twin-reversed arterial perfusion)Comparative cardiovascular developmental stages in different species
Developmental landmarks | Chick | Zebrafish | Mouse | Human |
---|---|---|---|---|
HH | Hpf | E | Days | |
Formation of single heart tube | 7–9 | 19 | 8 | 22 |
First myocardial contractions | 7–10 | 22 | 8.5 | 23 |
Heart looping | 11–13 | 33 | 8.5 | 24 |
First blood flow through heart | 10 | 26–28 | 9.5 | 24 |
Endocardial cushion formation | 13–22 | 48 | 9–11 | 28 |
Atrial septum, begins | 15–23 | n/a | 10–12 | 29 |
Ventricular septum, completion | 26–31 | n/a | 13–15 | 35+ |
3.1 Chick Embryo
At the onset of circulation, the chick embryo heart tube consists of three to five layers of myocardial mantle, enveloping a thick, cell-free layer of cardiac jelly, and a single cell layer of endocardium [8]. Within the individual myocytes, the contractile elements are in spatial disarray and only parts of them are functional [9, 10]. The early embryo heart and vasculature are devoid of nerve elements. The parasympathetic limb of the autonomic nervous system is the first to develop and is functional by HH stage 39 (development day 12) and the sympathetic by stage 42 (day 17) [11].
The initial period of heart formation is accompanied by rapid expansion of the extraembryonic bed. Hu and Clark measured the rate of growth of chick’s embryo ventricle from stage 12 to 29 and compared it to the combined weight of the embryo and its membranes. They noted a marked disproportion in growth rates between the heart and the rest of the embryo. At stage 12, the ventricle represents 4.5% of the total embryo mass and by stage 29, the ratio drops to 1.2%. During the same period, the weight of the ventricle increased by a factor of 30, while the mass of the embryo increased 120-fold [8]. While the relative weight of the ventricle decreases during this period, its work, paradoxically, increases [12]. The relative size of the embryo compared to the vitelline vascular bed is reflected in the distribution of cardiac output. At stage 18, some 80% of cardiac output is delivered to the extraembryonic vascular bed and by stage 24, with increasing growth and metabolic requirements of the embryo, the ratio drops to 65% [13].
Concomitant with growth there is a linear increase in heart rate of the developing embryo between successive stages [14]. During stages 12–29 stroke volume increases from 0.01 to 0.69 mm3 [8]. Burggren et al., found a relatively modest increase in heart rate of 100–130 bpm in stages 16–24 (day 3–4) but a fivefold attendant increase in stroke volume. Moreover, there was a “dramatic” 10 to 15-fold increase in cardiac output over the same developmental period. They suggest that the wide inconsistency in measured parameters clearly points to unidentified factors in our understanding of early embryonic cardiovascular dynamics [15]. In contrast to mature animals, where there is a reciprocal relationship between the size of the heart, the weight, and the size of the animal, a paradoxical relationship exists in the embryo, where an increase in weight is accompanied by increase in heart rate. In spite of the fact that the increase in heart rate markedly shortens the time of ventricular filling, there is a steady increase in stroke volume and aortic blood flow (cardiac output) [12].

Representative ventricular pressure curves from HH stage 16–24 chick embryos. (Adapted from ref. [17], used with permission of Wolters Kluwer Health)
Several studies comment on the invariant relation between the rate of embryo growth and cardiac output. During each developmental stage, there is approximately a doubling of aortic blood flow and stroke volume which, when normalized to embryo weight (without the embryonic membranes), remains constant. That is to say that across a 120-fold increase in embryo weight, from stages 12 to 29, the blood flow per milligram of embryo weight remains remarkably constant [8, 12, 14]. The wave propagation velocity, arterial pressures, and circulating blood volume are likewise tightly regulated and optimized within a narrow range across developmental stages [18]. Since the heart and embryonic circulation lack innervation and hormonal control, the obvious question arises, as to how does the heart “sense” and meet the changes in its own development and matches them with the specific metabolic demands of rapidly developing tissues, with such consistency? It has been proposed that the metabolic demands of the tissues directly regulate cardiac output [16]. Moreover, recent evidence suggests a tight link between the metabolism, angiogenesis, and endothelial factors controlling vascular growth [19], implying that, with respect to peripheral tissues, the heart plays only a secondary role in the overall embryonic hemodynamics. This remarkable, as yet unexplained matching between metabolic demands and blood flow clearly points to the periphery as the primary determinant of flow.
By applying the hydraulic reservoir model, i.e., the 3-element Windkessel, it is possible to estimate the arterial impedance (opposition) of vasculature, to steady and oscillatory flow. (See Fig. 16.9). The model is based on electrical analogue and assumes that pressure and flow are linearly related in the frequency domain. It allows for calculation of total, steady-state and oscillatory power generated by the ventricle, by simultaneous measurement of ventricular flow and pressure. According to this paradigm, the steady-state or mean power is the energy required by the heart to drive and maintain the flow and pressure. The oscillatory component of the total power, on the other hand, is expended on rhythmical expansion of vessels and is considered as “wasted” power spent on arterial pulsations [20, 21]. (The merit of this and other models of circulation will be discussed in Part II). Clark and Hu reported a dramatic increase in steady-state cardiac power of 350-fold! between stages 18 and 29. During the same developmental period, the peripheral vascular resistance, calculated from the ratio of stroke volume and vitelline artery pressure, drops by a factor of 5–24 [8, 12]. Lucitti et al. reported a more moderate increase in steady-state power by a factor of 8 between stages 21 and 27 [22], while Zahka et al. found a 27-fold increase between stages 18 and 29 [23].
Would the immature embryonic heart be able to sustain such extremes in workload within such a short time span? And if so, what are its limits and regulatory mechanisms?
3.2 Zebrafish Embryo
In zebrafish embryo, the individual myocytes begin to contract irregularly at 20 hours post-fertilization (hpf) (somite stage, S22) at the rate of about 25 beats/min. By 22 hpf (S26) when the first movement of blood is observed, the heart beats rhythmically at about 90 bpm with a corresponding shift of the pacemaker to the venous (caudal) end of the heart tube. As the heart begins to loop at 33 hpf, the frequency of contractions gradually increases from 140 to 180 bpm at completion of looping [6, 24].
By 48 hpf the heart consists of a smooth-walled tube, partitioned into four identifiable parts, namely, the sinus venosus, the atrium, the ventricle, and the bulbus arteriosus. The heart tube is lined with the endocardium and consists of a single layer of myocardium, except in the ventricle, where it is two or three cell-layers thick. The endocardial jelly is gradually resorbed from the ventricle, but can still be seen in the atrium and the bulbus [25]. The heart is covered with epicardium and lies in the pericardial cavity. There are no valves separating the atrium, the ventricle, and the aortic bulb at this stage. The valves appear only 3 days later, by day 5. The functional significance of the jelly now becomes more apparent, since, despite the presence of endocardial cushions, the unidirectional flow gives way to significant backflow between the atrium and the ventricle (72–96 hpf) [26]. Comparable to the ventricular growth pattern in the chick embryo, the zebrafish ventricle of a 48 hpf embryo represents 10.5% of its total weight and decreases to 1.8% in a fully grown animal [25].
Hu et al. have pointed out to the unique role of the aortic bulb (conus arteriosus) in zebrafish which, like in higher vertebrates, has initially a myocardial character, but becomes replaced by smooth muscle in the course of 4 weeks post-fertilization, when the animal matures. They suggest that the bulbus functions as a “capacitor,” maintaining a continuous rather than pulsatile flow of blood in the gill arches [25]. A similar mode of action has been described in chick embryos [17] and larval bullfrog embryos, where during early stages the aortic bulb generates pressures in excess of ventricular systole [27]. We shall see (Sect. 11.3) that a functional analogue of this organ can be found in a number of invertebrates and lower vertebrates, i.e., the lance let (Amphioxus), where berry-like dilations in the blood vessels, the so-called peripheral hearts, serve to temporarily dam-up the flow of blood and eject it into the downstream vessel at a higher pressure.

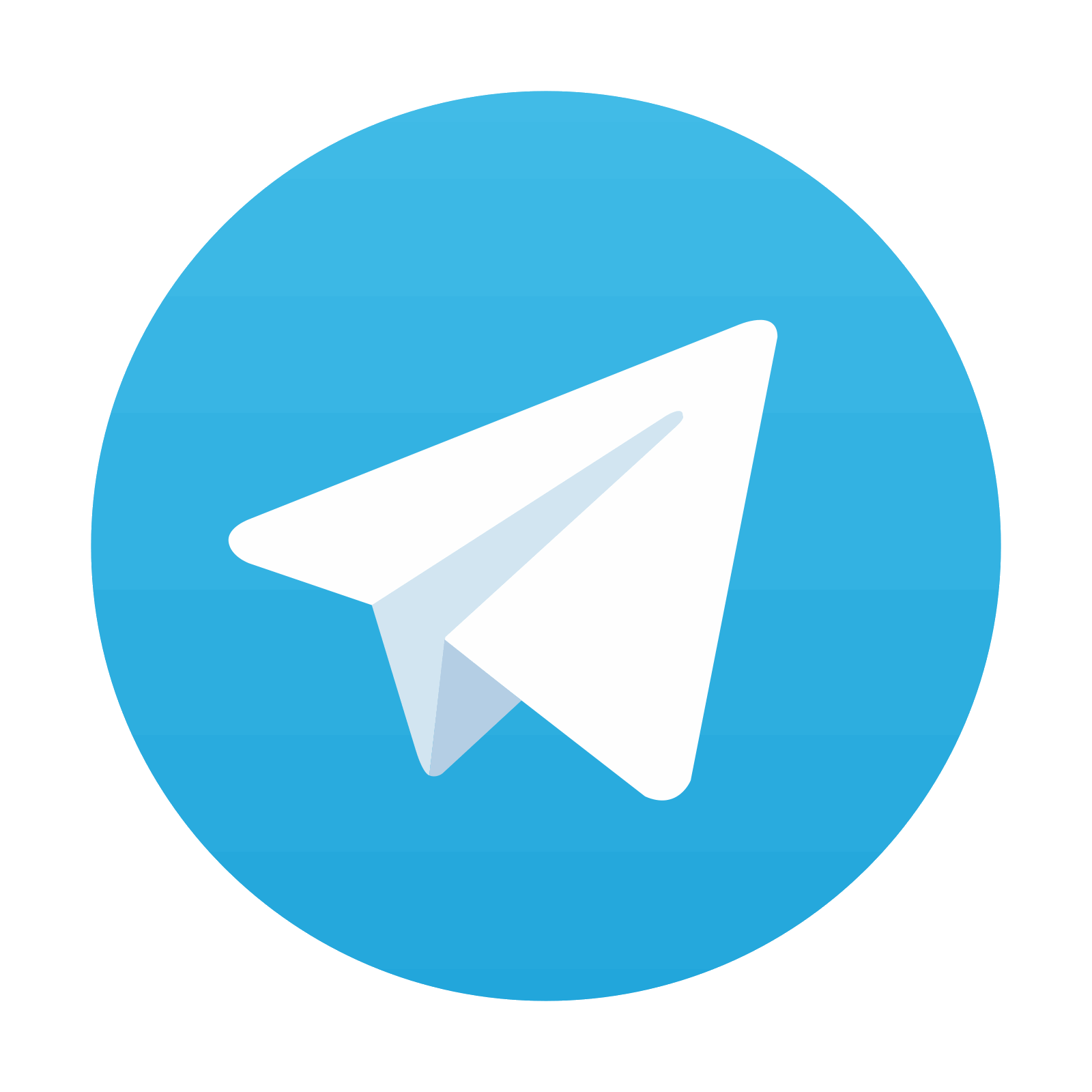
Stay updated, free articles. Join our Telegram channel
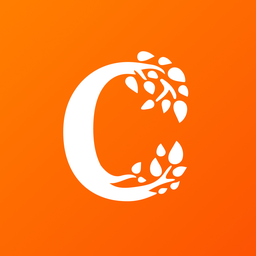
Full access? Get Clinical Tree
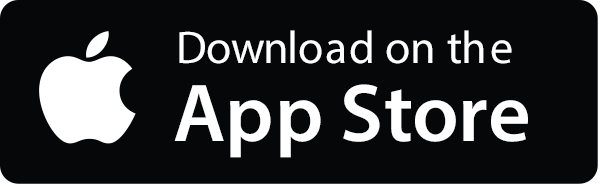
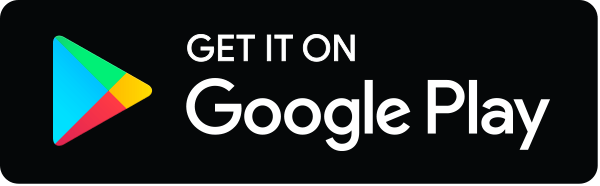