Keywords
Heart rate variabilityRespiratory sinus arrhythmiaChronocardiagramSynchrony of pulse and respirationOpen systemsSelf-organizationDissipative structuresCardiorespiratory phase synchronizationThermodynamic equilibriumActive fluidsTime organizationTime structuresBiological rhythmsMicrocosmic and macrocosmic rhythmsCardiovascular circadian clocksSpiral wavesLinear timeCyclical timeThreefold time organismCore body temperatureSleep–wakefulness cycleOne of the unintended consequences of introducing the isolated heart preparation into experimental physiology was a severance of the link between the respiratory and circulatory functions. With the adoption of the cardiocentric model by which the heart became a pump and the blood an “inert fluid,” the focus of research was directed mainly at the mechanical aspects of cardiovascular and pulmonary functions, while the interest in periodic phenomena as the actual carriers of vital functions proportionally waned (cf. Chaps. 15 and 16). This paradigmatic development led to a gradual decline in knowledge about the nature of time structures and periodical processes that were at the core of the medical doctrine well into the nineteenth century (cf. Chap. 22). A detailed review of periodic phenomena in humans is beyond the scope of this chapter, and only selected highlights related to respiratory and heart rate variability are given (cf. Refs. [1, 2] for review). Heart rate variability and its modulation by respiration are examined, and the importance of the ratio 4:1 for the overall health is discussed. The concept of “open systems” and “dissipative structures” in physics led to the discovery of self-organizing phenomena in biology and paved the way to an expanded view of homeostasis. Through internalization of external (macrocosmic) rhythms, the organisms have developed an inner time organization which tends toward greater autonomy as the organism ascends the evolutionary ladder. A hierarchy of endogenous (microcosmic) rhythms exists, ranging from milliseconds to hours, days (circadian rhythms), and beyond. In humans, they correspond to the threefold functional domains of the nerve-sense, cardiorespiratory, and metabolic systems.
23.1 Heart Rate Variability
Stephen Hales (1677–1761), the first to perform direct measurement of arterial pressure in animals, reported on beat-to-beat variation in synchrony with respiration [3]. Carl Ludwig’s (1816–1895) invention of a smoked drum kymograph which allowed for simultaneous recording of blood pressure in relation to respirations (1847), inaugurated a new era in chronobiological research. Ludwig’s observations of heart rate acceleration in a dog during inspiration, and its deceleration on expiration, formed the basis for what is known as “respiratory sinus arrhythmia” (RSA) [4]. A landmark discovery related to RSA was the invention of the electrocardiogram (ECG) by Willem Einthoven (1860–1927) in 1895. In addition to continuous recording of the heart’s electrical activity, it was now possible to accurately time the ECG signals with the respiratory cycle [5]. The introduction of a portable device for continuous, i.e., 24 h recording of the ECG by Norman Holter in 1961, significantly extended the range of monitoring and paved the way for diagnostics of cardiac arrhythmias in the context of diurnal cycles [6]. Among the numerous researchers who made contributions in the field of chronobiology was a group of physiologists headed by Günter Hildebrandt (1924–1999) whose seminal work on circadian rhythms laid the foundation for a “therapeutic physiology” [7, 8]. Finally, the advent of modern digital signal acquisition led to the development of computerized techniques of heart rate variability (HRV) in time and frequency domains and to the discovery of new methods based on nonlinear models.1 Exponential growth of studies in HRV prompted the Task Force of the European Society of Cardiology and the North American Society of Pacing and Electrophysiology to publish standards for quantitative measurement in physiological research and clinical application [9].

The effect of respiratory sinus arrhythmia on the R-R interval. Electrocardiogram in red, respiration in gray. Inter-beat interval, IBI (black double headed arrows); the time between two consecutive R-peaks in the ECG. Respiratory sinus arrhythmia (RSA), calculated as longest R-R interval during exhalation (L-IBI) minus the shortest heart period during inhalation (S-IBI). (Reproduced from ref. [12], used with permission of Journal of Cardiovascular Development and Disease)

Chronocardiogram of a sleeping subject. Time-compressed record of beat to beat intervals (horizontal axis) and amplitude (vertical axis) sampled over several hours at high frequency (4000 Hz), with Fourier transformation performed at 5 min intervals. Low amplitudes are color-coded blue, medium amplitudes in white, and high amplitudes in red. Periods of deep sleep are marked by regular coupling between heart rate and respiration (orange arrow), intervals of rapid eye movement (REM) sleep by chaotic rhythms (white and red). Predominant activity of the autonomic system is marked at the bottom of the record. Sympathetic activity marks a state of arousal, parasympathetic rest, and regeneration. (Reproduced from ref. [14], used with permission of IEEE)
Over the years the HRV has become a widely used risk assessment and prognostic tool. An example of clinical application of HRV is fetal heart rate monitoring in parturients introduced in the mid-1960s. The loss of fetal beat-to-beat variability has proven to be a relatively reliable indicator of fetal distress [15]. A variety of cardiovascular risk factors and disease conditions were later shown to be associated with changes in HRV. For example, reduced HRV is linked to increased morbidity and mortality in patients with acute myocardial infarction [16], diabetes [17], and heart failure [18] and has predictive value for complications in patients after cardiac surgery [19].

Schematic of autonomic cardiovascular control. The principal function of the autonomic nervous system (ANS) is to coordinate bodily function in response to changes in internal and external environment. Polarity of the ANS is expressed in its two divisions with seemingly opposing, but complementary functions. Simply stated, the sympathetic system prepares for “fight or flight” and the parasympathetic for “rest and digest.” Both branches of the ANS contribute to cardiovascular control. Parasympathetic preganglionic neurons send axons chiefly via the vagus nerve to the postganglionic neurons located in the ganglia of the heart. They secrete acetylcholine (AcC) which inhibits the discharge of authorhythmic (pacemaker) cells at the sinoatrial (SA) and atrioventricular (AV) node, causing bradycardia. Thoracolumbar sympathetic outflow supplies the sympathetic chain in proximity of the spinal cord and the paravertebral ganglia whose postganglionic fibers secrete norepinephrine (NE). Adrenal medulla is considered a sympathetic ganglion that secretes NE and epinephrine (Epi) directly into the blood stream. Sympathetic postganglionic fibers release NE at the pacemaker cells causing increase in heart rate (β1) and inotropy (increased ventricular contractility). Peripherally, vascular smooth muscles are almost exclusively innervated by the sympathetic fibers where NE causes vasoconstriction and decrease in blood flow. (Reproduced from ref. [12], used with permission of Journal of Cardiovascular Development and Disease)
In view of this impasse, we will consider autonomic control of HRV and related periodic functions that control cardiovascular homeostasis in the context of the emerging discipline of chronobiology [7, 23].
23.2 Synchrony of Pulse and Respiration

Rhythms of pulse, respiration, arterial pressure, and peripheral perfusion in healthy adult subjects during sleep typically assumes whole number values with ratio 4:1. (Adapted from ref. [29])

Heart rate variability during wakefulness and stages of sleep (lower panel). Note the synchrony of heart rate and respiration in deeper stages of sleep (orange arrow) and marked desynchronization during rapid eye movement sleep (REM) and wakefulness (red and green bars, respectively). Color coding of power spectrum is the same as in Fig. 23.2. (Reproduced from ref. [14] used with permission of IEEE)

A 24-h record of chronocardiogram, pulse respiration ratio and autonomic activity in a 11-year-old boy. Note phasic synchronization of pulse and respiration in sleep (orange arrow) oscillating around the mean value of 4.7 (middle panel). The lower panel demonstrates predominance of parasympathetic activity during sleep (regenerative phase, blue markings), and mostly sympathetic modulation during the day (red markings). (Reproduced from ref. [14], used with permission of IEEE)
Bartsch et al. investigated physiologic coupling between heart and respiration in different stages of sleep in 189 healthy individuals (99 females, 90 males, and ages ranging from 20 to 95). The results demonstrated a 400% increase in CRPS in regenerative deep sleep and confirmed that pronounced coupling between the heartbeat and respiration is not affected by the advanced age [32]. Although unrelated to sleep, cardiorespiratory phase coupling has been reported during isoflurane/nitrous oxide general anesthesia [33].
Ahn and coworkers employed a novel time-series analysis method to explore synchronization of cardiac and respiratory rhythms in a group of 20 healthy, young (ages 21–24), 20 elderly (ages 68–85) individuals without coronary artery disease (CAD), and 74 elderly patients (ages 68–85) with known CAD. The study showed that despite continuous fluctuations, cardiac and respiratory systems lock into a “highly specific temporal pattern” marked by frequent loss of synchrony (within a single breath) and its prompt recovery. (Healthy controls were resting but kept awake by watching an animated video.) All subjects demonstrated a pronounced synchrony between respiration and heartbeat with fixed ratios at 1:3 and 1:4 (three or four heartbeats for each respiration). In patients with coronary artery disease; however, CRPS occurred at lower ratios (2:1), suggesting that this group may be less adaptable to internal and environmental perturbations [34].
Similarly, Cabiddu and coworkers showed that CRPS is influenced by the overall state of health and is more pronounced in athletes [35] and in those who practice meditative and relaxation techniques [36]. Von Bonin and coworkers compared HRV (in high and low frequency domains) and the amount of CRPS in a group of 87 individuals between 35–50 years of age. They determined that longer periods of CRPS significantly correlated with perception of general health and resistance to stress, suggesting that CRPS is an indicator of quality of life [37]. Researchers from the same group have demonstrated that, in healthy volunteers, rhythmic speech exercises, i.e., classic meter poetry recitation,3 promote cardiorespiratory coupling lasting up to 15 min beyond duration of the exercise [38]. Given the fact that CRPS is profoundly disturbed in cancer patients and in patients with autonomic dysfunction (see [39] for refs.), guided speech therapy has become a promising therapeutic modality not only in tumor-related fatigue but also for adjuvant therapy in hypertensive patients [40].
23.3 An Expanded View of Homeostasis
The principal function of the respiratory and cardiovascular system is optimal delivery of oxygen and nutrients to the tissues and removal of metabolic products. Respiratory rate and cardiac output ultimately depend on immediate metabolic needs, be it at rest or at any level of activity. From an evolutionary perspective, greater metabolic demands are closely linked to the transition from water-based gill respiration to the development of lung respiration. In the process, the amphibian heart undergoes a radical transformation (addition of the left atrium) and subserves the pulmonary circulation. The mastery of gravitational environment attained by warm-blooded mammals and birds (where most chemical reactions speed up two- to threefold for every 10 °C in temperature) calls for a far greater oxygen extraction and delivery made possible by the highly efficient regulatory system that allows for rapid uptake of oxygen and release of CO2 by the blood (cf. Fig. 11.2). The heart as an organ of flow-restraint is now placed at the juncture of the phylogenetically older systemic and the developmentally “younger” pulmonary circulations, maintaining the ratio of flows close to unity (QS: QP = 1:1; cf. Chap. 19). The heart is therefore called upon to synchronize respiratory as well as metabolic rhythms, the inner (microcosmic) and outer (macrocosmic) environments (cf. Sect. 23.5). That this is indeed likely the case has been demonstrated by mathematical modeling that optimal coupling of HR and respiration (CRPS) is an energetic optimization (saving around 3% of energy over a single breath) [41].
The notion that virtually all biologic phenomena are subject to a hierarchy of rhythms (see Sect. 23.5) is yet to find its way into the standard texts of physiology. For example, in a recent editorial, West argued that the explanatory power of cardiovascular and respiratory control based on traditional control theory falls short in face of the richness and complexity of non-equilibrium phenomena and proposed that the discovery of nonlinear dynamics and chaotic systems in physiology can be compared to the transition from the industrial to information age [22]. The case in point is the inability of linear models to uncover the underlying lawfulness between HR and respiration synchronization (CRPS). Considering the above, let us briefly examine some emerging concepts that broaden the scope of homeostasis.
23.3.1 Open Systems in Physics and Biology
It has long been recognized that physiological processes within organisms are not merely a continuation of physical-chemical forces (laws of interactions, properties, and relationships of matter) active in inorganic nature but occur within the confines of a finely controlled inner environment. Should these entropic chemical-physical forces gain the upper hand, the organism is subject to death and disintegration. In a sense, life can be viewed as continuous overcoming of inimical environmental conditions which, paradoxically, serve as a fulcrum for evolutionary development. It was internalization and maintenance of the primordial aquatic milieu that spurred the emergence of higher organisms. What early marine organisms obtained from their watery habitats is now functionally internalized [42]. Claude Bernard (1813–1878) was the first to suggest that extracellular fluid provides a stable inner environment essential for optimal tissue and organ function. In response to claims that life could be reduced to chemistry and physics, Bernard responded, “Organic individual compounds, though well defined in their properties, are still not active elements in physiological phenomena. They are only passive elements in the organism,” because, he maintained, “The living organism does not really exist in the milieu extérieur, but in the milieu intérieur. A complex organism should be looked upon as an assemblage of simple organisms…that live in liquid milieu intérieur” (cited in Ref. [43]).
Expanding on Bernard’s work, Walter Cannon (1871–1945) introduced the concept of “homeostasis” as the process by which the “fluid matrix” of the organism establishes and maintains an internal functional stability in the face of environmental perturbations [44]. A stage was set where linear models were gradually replaced by “operational loops” dressed in tecnomorphic language such as feedback, set point, signal, redundancy, etc.
This relatively static, cybernetic view of biological regulation was expanded by Bertalanffy and others who, to bridge the rift between the physical-chemical laws operative in the outer environment and the organism’s capacity to “self-organize” according to higher lawfulness, proposed a theory of open systems in medicine and biology [45], one of the principal tenets of the modern systems biology. Bertalanffy’s work followed in the wake of the introduction of open systems physics by Ilya Prigogine (1917–2003, awarded Nobel Prize in chemistry for discovery of dissipative structures in 1977). Prigogine pointed to a profound difference between equilibrium and dissipative processes . Isolated (closed) systems evolve toward thermodynamic equilibrium, a condition where physical and chemical properties do not change with time. Examples of isolated systems are internal combustion engines, or the growth of a crystal, which in its final form attains a time-independent state of equilibrium. According to the second law of thermodynamics, such a system attains a state of maximal entropy (wasted heat) and minimum free energy (potential energy to perform more work).

Examples of dissipative and non-dissipative structures. Rayleigh–Bénard convection cells (a) occur due to gradients in temperature and density in a thin layer of heated fluid (silicon oil). The cells assume a hexagonal vortex form in heated silicon oil. Bénard convention instabilities are examples of self-organizing dissipative structures. A cluster of hexagonal quartz crystals (b), an example of fixed, non-dissipative form. (a, Reproduced from ref. [47], used by permission of J.S. Haupt)
It is important to note, however, that self-organized activity is fueled by a constant supply and removal of materials (and energy) which maintains the system away from thermodynamic equilibrium, thus preventing it coming to a halt. Under such “unstable” conditions, local fluctuations may be amplified and “invade” the entire system. Significantly, attainment of this dynamic state, facilitated by metal catalysts and biological enzymes, is paradoxically linked to a minimization of energy and an increase in entropy, a manifestation of the second law of thermodynamics [48].
Finally, there is the question of causality of forms of crystals and self-organizing dissipative structures such as flames of candles, Rayleigh–Bénard cells, and tornadoes. Addressing this issue, S. Kellert, an authority on fractals and chaos theory, compares the mechanical causation which he holds to be causal, with geometrical causation which he believes to be responsible for “common patterns or regularities at a more macroscopic level of analysis” and in view of which it is meaningless “to try to understand the behavior of the whole system by tracing each individual process. Instead, one needs to find a way of representing what the system does on the whole or on average, which abstracts from such specific causal detail” [49].
23.3.2 Self-Organization

Spiral waves in three dimensional medium. Right anterior oblique (image on left) and left anterior oblique (image on right) views of the right atrium. The sinus node (red) generates depolarizing waves spreading radially in all directions. Fields of equal color represent times of simultaneous activation (isochrones). Red—early activation, purple—late activation. SVC superior vena cava, IVC inferior vena cava, SA sinoatrial node, TR tricuspid, AV atrioventricular node. (Courtesy of Heather DeBrita, Carto Systems)
However, there is a marked difference between the self-organizing phenomena in physics and chemistry and the events taking place in an organism where the self-organization process runs in a twofold sense. What must be supplied externally in the case of a physical or a chemical self-organizing system, either through constellation of favorable circumstances in nature or artificially, e.g., in a laboratory, must be self–accomplished in an organism internally. First, the organism must self-organize the formation of physical organs, i.e., structural proteins, enzymes, cells, organ systems, etc., that will serve as a starting point for the emergence and maintenance of the secondary, self-organizing physiological systems that will execute and uphold homeostasis. According to Heusser, over and above the first self-organizing formative principle that organizes an organism in space, rules a higher formative and functional principle organized in time [53].
The concepts of open systems and self-organization provide yet another argument in support of our thesis, namely, that the blood is not moved by the pressure gradient between the left ventricle and the right atrium, but because of chemical (i.e., thermodynamic) disequilibrium between the metabolically active organs and the vascular endothelium/red blood cell interaction locally, and the pulmonary and systemic circulations globally (cf. Chap. 11). In fact, self-driven flows (i.e., without pressure gradients) are a well-described phenomenon in physics of “active fluids.” A recently studied example involves kinesin clusters or “molecular motors,” consisting of microtubules and ATP, both widely distributed in eukaryotic cells. When confined to cylindrical channels, the consumption of ATP by kinesin clusters creates large-scale vortices that spontaneously organize into directional flow. Such active fluid systems can propel themselves the length of up to 1 m at velocities of 10 μm/s [54], well within the range of RBC movements in the capillaries. Commenting on the nature of such self-driven flows, Morozov noted that, “The active fluid was not driven from outside, but self-organized the random motion of its individual microtubular bundles into coherent, unidirectional flow” [55]. Given that ATP is actively released by the RBCs during passage through tight endothelial channels (cf. Sects. 17.1.1 and 21.2.1), it is conceivable that such non-pressure-driven flows also exist in the microcirculation [56]. In the context of open-system dynamics, capillaries are the peripheral channels where a state of energetic disequilibrium between the RBCs and the endothelium is actively maintained. The subtotal of this energetic disbalance translates into suction and pressure that can be measured at various points of the cardiovascular system.
23.4 Linear Time, Cyclical Time, and Consciousness
Parallel to the evolution of human consciousness, the experience and concept of time have undergone a radical transformation. In contemporary terms, time, known as the absolute or Newtonian time , progresses independently of observer at constant pace throughout the universe. It is imperceptible and of abstract, mathematical nature. Ancient Greeks, for example, distinguished between two different kinds of time: chronos, the numeric or chronological time, and kairos which represented its qualitative aspect—the “right” or opportune time. For Aristotle, time was inherently bound to movement which can proceed at different rates (faster or slower) and requires the presence of a witness; a soul or psyche capable of experiencing the change [40]. The Greeks, moreover, perceived time as following in cycles by which the universe is born and dies away [57].5
In classical (Newtonian) mechanics where processes are determined and can proceed forward as well as backward, the concept of time is employed merely as a geometric parameter for following a succession of dynamic events. Here, time is “reversible,” and past and future play an equal role. This gives the impression that time consists of reproducible, equal parts (time quanta). In far-from-equilibrium systems, on the other hand, time is an integral part of self-organizing events arising from the chaotic systems described above. The hallmark of these time-bound processes is directedness or the “arrow of time” and hence irreversibility.6 It is precisely due to this irreversibility that chaotic systems have the possibility for bringing forth order out of chaos [59]. In other words, chaotic structures are the means whereby a non-temporal, non-material content or information can be imprinted on a physical substrate conveying form and meaning (cf. Sect. 25.4). According to Prigogine, the chaotic structures are “points of transition from potentialities to actualities” [60].
Thus, in addition to having a form–in-space, an organism also possesses a time-Gestalt or a form-in-time of higher order. When the momentary changes in form are known, e.g., the growth process of an embryo or a plant, one can reconstruct the entire formative process in-time, say by time-lapse photography. Of note, the common characteristics of an organism such as growth, nourishment and excretion, reproduction, breathing, regeneration, wound healing, apoptosis, and rest-activity cycles, to name a few, are all organized in time. Their temporal compartmentalization makes it possible to run unhindered in the limited space of an organism. The movement of blood, lymph, and other bodily fluids is therefore regulated by a highly differentiated time matrix or “time body” subject to cycles, repetitions and reversals that go beyond the conventional, linear time. Periodic time (rhythm) is the medium in which evolves the stream of life.
How do we experience time? As mentioned, nuances and qualities of time can only be experienced by consciousness which, however, extends beyond time. Animal life is inextricably bound to the flow of time with consciousness directed either to perception of its own body or to perception of the surroundings. Animal’s consciousness, i.e., soul life, however, is directed at the fulfillment of instinctual impulses and drives aimed at self-preservation. Humans, too, can give themselves to outer or inner impressions, but can also separate from the stream of time. Time becomes void, timeless, a no-thing. One can willingly turn attention to the past (memories),7 experience the present, or think about the future. Rohen maintains that under certain circumstances time is suspended and loses its identity: “We may experience a great deal in a split second of intense experience or have very few significant experiences (or even none at all), for hours at end” [62].
It is recognized that consciousness and related qualitative experiences necessarily point to first person (subjective) observation, while neuronal processes can be quantified only via third person (objective) observation. The question arises, how does one transition from neurobiological to psychological phenomena? Voluminous literature on the subject of bridging the mind/body gap in domains of neurosciences exists, but this subject is well beyond the scope of this chapter. Let us only mention that models based on the synthesis of rhythmical (or functional) and neuronal processes are increasingly gaining ground (see [63] for further refs.) Weger and Edelhäuser advance the view (originally proposed by Steiner [64]) that rather than generating, the brain mirrors lawful thought patterns into individuated conscious form. Accordingly, the oscillating neurons, neuronal pathways and portions of the brain, simultaneously represent the material as well as the formal8 (non-material or qualitative) aspects of reality. An important point of this theory, in the context of our discussion, is the fact that the common denominator between the physical/neuronal and mental (formal)/conceptual phenomena is rhythm. Thus, in rhythm, the non-spatial, non-temporal aspect of reality is joined with the material substrate to become an inner experience [63]. Rhythmical patterns of neuronal and brain activity are signatures of these events.
23.5 Macrocosmic and Microcosmic Rhythms
Androsthenes, Alexander the Great’s admiral during the military expedition to India in the fourth century BCE, was the first to document observations of daily opening and closure of leaves on a tamarind tree. Almost two millennia later (1729), French astronomer de Marian reported that diurnal movements of mimosa leaves continue for several days, even if the plant is kept in the dark, suggesting that it possesses an internal clock. Formal study of diurnal rhythms in organisms began with Carl von Linné’s publication of “Philisophia Botanica” in 1751. William Ogle was the first to report on periodic swings of temperature in humans in 1866, at the time when his contemporary Charles Darwin studied rhythmical phenomena in animals and plants in relation to evolutionary processes. Darwin observed that the tips of grass seedlings respond to changing light conditions and proposed that daily movements of leaves have a survival value [23].

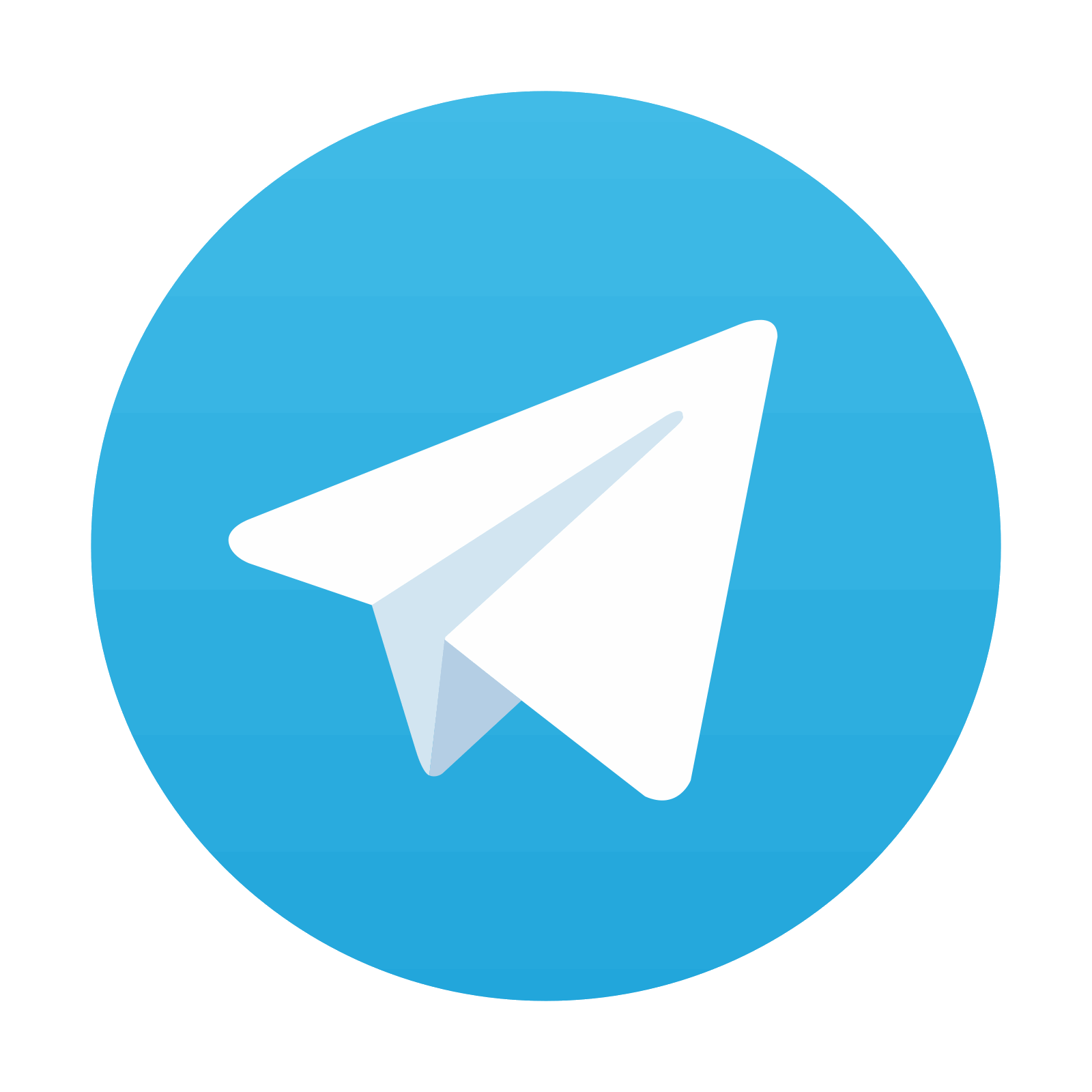
Stay updated, free articles. Join our Telegram channel
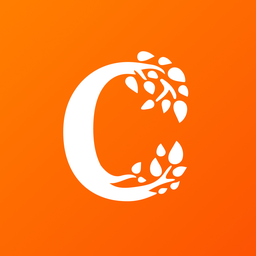
Full access? Get Clinical Tree
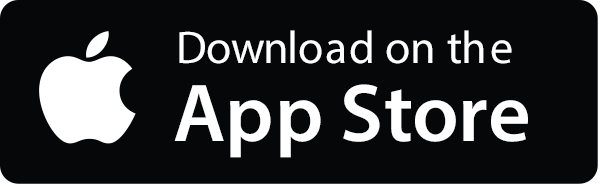
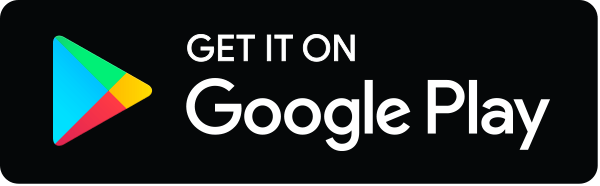