Outline
Myocardial Extracellular Matrix Structure and Composition, 63
The Myocardial Fibroblast, 63
Myocardial Extracellular Matrix Proteolytic Degradation: The Matrix Metalloproteinases, 64
Transcriptional Regulation of Extracellular Matrix Degradation; Matrix Metalloproteinase Polymorphisms, 64
Matrix Metalloproteinase Posttranscriptional Regulation, 66
Matrix Metalloproteinase Posttranslational Regulation, 67
Endogenous Matrix Metalloproteinase Inhibition, 67
Heart Failure With Reduced Ejection Fraction and Extracellular Matrix Remodeling—Myocardial Infarction, 68
Heart Failure With Reduced Ejection Fraction and Extracellular Matrix Remodeling-Dilated Cardiomyopathy, 69
Heart Failure With Reduced Ejection Fraction and Extracellular Matrix Remodeling—Hypertensive Heart Disease, 70
Myocardial Extracellular Matrix Remodeling in Heart Failure—Diagnostic Potential, 71
Imaging, 71
Summary and Future Directions, 74
Acknowledgments
Dr. Francis Spinale is supported by the Research Service of the Department of Veterans Affairs. The author wishes to recognize the significant contributions of past MUSC and USC medical and graduate students that participated in our cardiovascular research program over the past two decades.
Extracellular matrix (ECM) remodeling in the context of heart failure (HF) is not readily described by a specific pathological stimulus, but rather the downstream consequence of multifactorial events. One useful categorical description that encompasses both the HF presentation and the key underlying physiological manifestations has emerged. Specifically, in patients with an HF presentation whereby quantitative imaging reveals a reduced left ventricular (LV) ejection fraction (EF <50%), this has been assigned the definition of HF with reduced EF (HFrEF) ( see Chapter 37 ), whereas the same clinical presentation accompanied by a relatively “preserved” EF (>50%) has been assigned the definition of HF with preserved EF (HFpEF) ( see Chapter 39 ). In general terms, the pathophysiological disease states that cause predominant and significant myocardial injury and loss of contractile units, such as ischemic heart disease and the dilated cardiomyopathies, would likely give rise to HFrEF. In contrast, HF that arises from an increase in long-standing LV afterload, such as hypertension or aortic valve disease, would be examples that give rise to HFpEF. It must be emphasized that in both categorical conditions, the underlying myocardial disease process is not the definition of HF, but only when they are sufficient to produce clinical symptoms do these definitions apply. In addition, these HF phenotypes are not mutually exclusive; thus patients may present with features of both impaired systolic function and diastolic function. Nevertheless, recent clinical trials have identified that a specific pharmacological intervention can be effective for HFrEF, but yield only equivocal results for HFpEF, underscoring the concept that the underlying structural basis for these HF phenotypes are distinctly different. Therefore, for the purposes of focus, the prototypical example of HFrEF as it applies to myocardial infarction (MI) and dilated cardiomyopathy (DCM) ( see also Chapter 20 ), and the prototypical example of HFpEF as it applies to hypertensive heart disease, will be utilized in this chapter.
One of the key features of HF irrespective of the category is that changes in LV, myocardial structure, and geometry occur and have been generically termed LV remodeling ( see also Chapter 12 ). Importantly, there are key features of the LV remodeling process that are present in either HFrEF or HFpEF and not only hold prognostic relevance but also provide potential clues to the underlying biology unique to each of these HF phenotypes. LV remodeling can be defined as changes in the geometry and function of the LV, which in turn is a summation of cellular and extracellular events. Moreover, it has become increasingly evident that the myocardial ECM is not a static structure, but rather a dynamic entity that may play a fundamental role in myocardial adaptation to pathological stress and thereby facilitate the remodeling process. A greater appreciation for the highly complex and dynamic nature of the ECM can be realized by myocardial imaging and direct interrogation of the interstitium, and will be discussed in upcoming sections. In both human and animal studies, it has been reported that alterations in the collagen interface, both in structure and composition, occur within the LV myocardium, which in turn may influence LV geometry. Moreover, dysregulation of key translational and posttranslational control points with respect to ECM have been directly linked to adverse LV remodeling and will be examined in this chapter. Therefore identification and understanding of the biological systems responsible for ECM synthesis and degradation within the myocardium hold particular relevance in the progression of both HFrEF and HFpEF. Accordingly, the purpose of this chapter is fourfold. First, we present a brief overview of myocardial ECM structure and biosynthesis. Second, we briefly demonstrate how the ECM is altered in specific disease states that give rise to HFrEF (MI, DCM) and HFpEF (pressure overload), and integrate studies identifying alterations at the genetic level. Third, we present a summation of how measuring dynamic changes in ECM remodeling through either plasma profiling or imaging can provide diagnostic/prognostic utility in HF. Fourth, we examine where the field of ECM biology may be moving in terms of developing therapeutics.
Myocardial Extracellular Matrix Structure and Composition
The myocardial ECM contains a fibrillar collagen network, a basement membrane, proteoglycans and glycosaminoglycans, and bioactive signaling molecules. The myocardial fibrillar collagens, such as collagen types I and III, ensure the structural integrity of adjoining myocytes, provide the means by which myocyte shortening is translated into overall LV pump function, and are essential for maintaining the alignment of myofibrils within the myocyte through a collagen-integrin-cytoskeletal-myofibril relation. While the fibrillar collagen matrix was initially considered to form a relatively static complex, it is now recognized that these structural proteins can undergo rapid degradation and fairly rapid turnover. Collagen fibril formation entails posttranslational modification. The carboxyterminal of the procollagen fibril is cleaved by a proteolytic reaction that results in a conformational change necessary for collagen fibril cross-linking and triple helix formation. A critical step in the proper formation and structural orientation of the fibrillar collagen matrix is collagen cross-linking. Interruption of collagen cross-linking has been clearly demonstrated to alter myocardial ECM structure and in turn LV geometry and function. Furthermore, alterations in fibrillar collagen cross-linking have been identified in myocardial samples taken from patients with end-stage HF. While the newly formed, uncross-linked collagen fibrils are vulnerable to degradation, the triple helical collagen fiber is resistant to nonspecific proteolysis, and further degradation requires specific enzymatic cleavage. During collagen cross-link formation, the carboxyterminal peptide is released into the vascular space. Collagen type I fiber formation results in the release of a 100-kDa procollagen type I carboxyterminal propeptide (PIP). Similarly, the formation of mature collagen III fibers results in the release of a 42 kDa procollagen peptide (PIIIP). As will be discussed, a number of associated proteins are necessary for the proper transport, assembly, and stability of the collagen fibril, and monitoring these collagen peptides and associated proteins has been demonstrated to provide diagnostic utility in terms of ECM remodeling and HF progression.
The integrins are a family of transmembrane proteins that serve multiple functions with respect to myocardial structure and function. The integrins form the binding interface with proteins comprising the basement membrane and therefore directly influencing myocyte growth and geometry. Moreover, the integrins coalesce at important structural sites within the myocyte, called costameres, which are composed of cytoskeletal proteins, such as alpha-actinin and vinculin, which form a key intracellular support network for contractile protein assembly and maintaining sarcomeric alignment. The myocyte costamere is also where the integrins appear to cluster and interdigitate with an intracellular signaling cascade system, such as focal adhesion kinase. Thus, disruptions of normal integrin–ECM interactions will likely result in significant changes in myocyte structure and function.
There are a number of extracellular proteins that comprise the basement membrane, such as collagen IV, fibronectin, and laminin. Thus, while fibrillar collagen has been the focus of most past studies, both basic and clinical, collagen is actually a modest component of the ECM. Thus, studies that evaluate both collagen content as well as other constituents of the ECM are beginning to emerge. For example, patients with DCM and mechanical assist devices demonstrate marked shifts in both fibrillar collagen and the basement membrane component, laminin. Representative images from this study are shown in Fig. 4.1 . It is the basement membrane that forms the anchoring points for the fibrillar matrix and contact points for other proteoglycans, such as chondroitin sulfate within the ECM. For example, the abundant binding of negatively charged unbranched glycosaminoglycans within chondroitin sulfate results in a molecule with a high osmotic activity. Accordingly, changes in the content and distribution of these proteoglycan will affect hydration within the extracellular space, and in turn directly influence myocardial compliance characteristics. Moreover, these highly charged molecules within the ECM result in the formation of a hydrated gel that serves as a reservoir for signaling molecules and bioactive peptides. Therefore, the ECM is an important determinant of extracellular receptor–ligand interactions. Another important function of the myocardial ECM is that it serves as a reservoir for critical biological signaling molecules that regulate myocardial structure and function. For example, tumor necrosis factor (TNF) is initially a membrane-bound molecule that requires proteolytic processing and release into the interstitial space in order to form ligand complexes with cognate receptors. Another example is transforming growth factor (TGF)-β, which exists in a latent form bound to the ECM, and requires proteolytic processing within the myocardial interstitium to become a competent signaling molecule. TGF signaling produces multiple cellular responses—most importantly, the stimulation of ECM protein synthesis. Thus, while this chapter will primarily focus upon the collagen fibrillar network, it is becoming recognized that the myocardial interstitium is a complex environment, which contains structural and signaling molecules that directly affect overall myocardial form and function.

The Myocardial Fibroblast
The most abundant cell type within the LV myocardium is the fibroblast. This is the predominant cell type that is responsible for maintaining ECM homeostasis in terms of collagen synthesis and degradation. This does not imply that the fibroblast is operative in isolation, as significant biomechanical and bioactive signals between the cardiocyte-fibroblast and endothelial cell–fibroblast interactions likely dictate the rate and type of ECM synthesis and degradation. What appears to occur in disease states, whereby enhanced fibrosis and/or ECM remodeling occurs, is a significant change in the fibroblast phenotype. This process, whereby a significant population of phenotypically differentiated fibroblasts arises with ECM remodeling, can been termed as a transdifferentiation process, and the resultant cell type has been defined as the myofibroblast. However, this does not imply that the normal fibroblast phenotype is extinguished, but rather there is likely increased proliferation of fibroblasts that do not express the myofibroblast phenotype. Thus, in ECM remodeling there is likely expansion and proliferation of both fibroblasts and myofibroblasts, which in turn will significantly affect the balance between collagen synthesis and degradation. In terms of myocardial ECM remodeling in HFrEF, changes in the synthesis-degradation axis occur, resulting in ECM instability ( Fig. 4.2 ). In contrast, with HFpEF, robust expansion of myofibroblasts occurs, resulting in increased ECM synthesis and accumulation ( see Fig. 4.2 ). The specific cell type of origin with respect to the myofibroblast remains unclear and may arise from a stem-cell type, pericyte, or a clonal expansion of endogenous fibroblasts. Whatever the case, the myofibroblast is not found in normal LV myocardium but is readily identifiable in the context of LV remodeling. The myofibroblast is typically identified through cellular constituents and by function. Specifically, the mature myofibroblast expresses alpha smooth muscle actin (SMA), whereas normal fibroblasts do not. In addition, cultured myofibroblasts demonstrate much higher proliferation, margination, invasion, and ECM contractile behavior.

One of the more active areas of research in the cancer field is that of mesenchymal cell transdifferentiation (MET), whereby mesenchymal cells under control of the local environment will transdifferentiate into a proliferative cancer type. There are significant similarities in the signaling pathways that are evoked during this mesenchymal transdifferentiation process to that of fibroblast–myofibroblast transdifferentiation. Since the myocardial fibroblast is a cell type of mesenchymal origin, it follows that the expression of specific transcription factors and cell markers would be operative similar to that of MET. There is compelling evidence to suggest that this fibroblast transdifferentiation is accompanied by an intermediate step or cell type, the proto-myofibroblast. This proto-myofibroblast will express unique cell markers, such as a splice variant of fibronectin—the extra-domain A (fibronectin ED-A). What is clear is that the canonical thought that the fibroblast is a single phenotypic entity must be revisited, and changes in the type, proliferation, and function of sub populations of fibroblasts likely contribute to specific forms of ECM remodeling, and in turn will influence myocardial form and function with HF.
Myocardial Extracellular Matrix Proteolytic Degradation: The Matrix Metalloproteinases
The matrix metalloproteinase (MMP) are a diverse family of zinc-dependent proteases that play a role in normal ECM turnover as well as in pathological tissue remodeling processes. Currently, over 25 distinct human MMPs have been identified and characterized. While initially thought to only cause ECM proteolysis, a highly diverse set of biological functions have been identified, which include cytokine processing and activation of pro-fibrotic signaling molecules. Moreover, MMP activity is highly dynamic within the human myocardial ECM. Specifically, using microdialysis techniques coupled with MMP fluorescent substrates, it has been identified that continuous MMP activity exists within the human myocardium under ambient conditions, which can increase significantly in a matter of seconds. The MMPs were historically classified into sub groups based upon substrate specificity and/or structure, and an informal nomenclature for some of the individual MMPs arose from these initial substrate studies. However, there is significant overlap in MMP proteolytic substrates, and a more rigid numerical classification is now utilized. Important MMPs in the context of myocardial ECM remodeling include the collagenases, such as MMP-1 and MMP-13; the stromelysins/matrilysins, which include MMP-3/MMP-7; the gelatinases, which include MMP-9 and MMP-2; and the membrane-type MMPs (MT-MMPs). Taken together, once the MMPs are activated, these enzymes can degrade all ECM components; therefore it is important that the activity of these enzymes is kept under tight control and interaction. The regulation of MMPs can take place at the level of transcription, posttranscription, posttranslation, and endogenous inhibition. These levels of control are briefly outlined in the next few paragraphs with examples of how this regulation is operative in the context of myocardial ECM remodeling.
Transcriptional Regulation of Extracellular Matrix Degradation; Matrix Metalloproteinase Polymorphisms
Transcriptional regulation of MMPs is primarily determined by upstream gene promoter activity whereby a number of intracellular signaling factors bind to specific sequences within the MMP promoter sequence. As such, there has been considerable interest in nucleic acid substitutions (i.e., polymorphisms) that occur within the MMP promoter regions and in relation to overall MMP levels, and most importantly in relation to cardiovascular outcomes. There have been a number of MMP polymorphisms identified in key MMP types, which include the collagenases (MMP-1, MMP-8), the gelatinases (MMP-2, MMP-9), and stromelysins (MMP-3). A brief synopsis of MMP polymorphisms with respect to cardiovascular remodeling processes and selected citations are provided in Table 4.1 . This summary table is by no means exhaustive but underscores the fact that several polymorphisms, primarily within the MMP promoter regions, have been identified and associated with subsets of patients at risk for cardiovascular events.
Polymorphism | Biologic Effect | Clinical Association | References |
---|---|---|---|
MMP-9 | |||
-1562 C/T | Promoter activity | Post-MI remodeling | 1, 2, 19–22, 87, 90 |
Remodeling in DCM | 3 | ||
-279 R/Q | Catalytic activity | Remodeling in DCM | 1, 3 |
Risk of post-MI remodeling | 86, 91 | ||
Differing pharmacologic response in CVD | 88 | ||
Promoter Activity | CAD | 86, 101 | |
TAA/AAA | 100, 103 | ||
MMP-2 | |||
-790 T/G | Transcription factor binding | CAD, HF, CVD, HTN, TAA | 23, 24, 92–94 |
MMP-3 | |||
1171-5A/6A | Promoter activity | Post-MI remodeling | 4-7, 11, 22, 87, 96 |
1612-5A/6A | MVP | 97 | |
Remodeling in DCM | 4, 89 | ||
Promoter | CAD | 100, 104 | |
HTN | 104 | ||
AAA | 105, 106 | ||
MMP-1 | |||
-1607 1G/2G | Transcription factor binding | Risk of post-MI remodeling, HF, CVA | 9, 10, 89, 108 |
-519-340 A/G-C/T | Promoter activity | Risk of post-MI remodeling, BAV | 12, 98, 99 |
MMP-8 | |||
Promoter | CVD | 107 | |
TAA | 107 | ||
MMP-12 | |||
Promoter | CVD | 88 |
Several of the MMP types identified in Table 4.1 are associated with acute/chronic inflammation in that these proteases are expressed by inflammatory cells as well as being induced by mediators of inflammation, such as through cytokine signaling. For example, MMP-8 and MMP-9 are highly expressed by neutrophils and macrophages, and rapid induction of these MMP types through inflammatory signaling pathways has been well established. Coronary artery disease, and more specifically vulnerable coronary plaques, has been associated with inflammation and local activation of MMP-9 and MMP-8. With respect to MMP-9, a variant has been identified, which is a single base substitution between cytosine (C) and thymidine (T). The T allele results in a higher relative level of promoter activity when compared to the C allele. The presence of the MMP-9 T allele resulted in increased plasma levels of MMP-9. A naturally occurring variant in the MMP-3 promoter region are the 5A and 6A alleles; the 5A allele has been associated with increased MMP-3 promoter activity, and in turn, increased relative MMP-3 protein levels. In a study performed in over 2800 Japanese patients, the 5A polymorphism was associated with increased risk of MI, particularly in women. Mizon-Gerad and colleagues reported that homozygosity for the 5A allele in patients with nonischemic cardiomyopathy was associated with poor survival. In a preliminary clinical study, the addition of a guanine within the MMP-1 promoter region (-1607 1G/2G) was associated within an acceleration of adverse LV myocardial remodeling in patients post-MI. With respect to MMP-8, increased local MMP-8 levels and polymorphisms within the MMP-8 promoter region have been identified with coronary plaque progression and acute events. For example, Salminen and colleagues performed a genome-wide association study (GWAS) to that of plasma MMP-8 levels as well as overall cardiovascular events in a large cohort of patients. These investigators identified that in certain subsets of patients, polymorphisms within the MMP-8 promoter region were associated with different plasma MMP-8 levels. Past studies have identified that polymorphisms within the MMP-9 promoter region would also result in higher plasma MMP-9 levels and in turn impart increased risk for cardiovascular events. More importantly, however, Salminen and colleagues identified several important polymorphisms distal to that of MMP-8 itself using a GWAS approach: that of complement factor-H and in a specific member of the S100A family, both of which directly or indirectly may have influenced MMP-8 levels. While this is an oversimplification, these findings add to the body of evidence regarding an inter relationship between inflammatory signaling pathways, MMP induction, and cardiovascular risk.
Past studies of MMP polymorphisms and cardiovascular risk have identified both gender and ethnicity as important independent confounding variables in predictive cardiovascular risk models. Indeed, a past study reported that polymorphisms contained within the MMP-8 promoter region conferred increased risk for the progression of carotid artery disease, specifically in women. In another study, the severity of carotid artery disease was most strongly associated with a common MMP-9 polymorphism (-1562T) in women. With respect to ethnicity, specific MMP polymorphisms have been identified to confer increased risk of cardiovascular disease progression and cardiovascular events in Asians and in blacks of African descent. For example, a polymorphism within the MMP-9 promoter is more frequent in African-Americans and is associated with higher MMP-9 plasma levels. Since MMP-9 has been implicated to promote adverse cardiovascular remodeling, then this would imply a higher relative risk for disease progression with this MMP-9 polymorphism.
Specific MMP polymorphisms confer additive risk for cardiovascular events in subjects with other risk factors, such as diabetes and obesity. For example, MMP-9 polymorphisms identified in patients with metabolic syndrome appear to be synergistic with respect to cardiovascular events. Of potentially greater significance, MMP-2 and MMP-9 polymorphisms, which have been associated with cardiovascular disease progression ( see Table 4.1 ), have been more commonly identified in childhood obesity. While remaining speculative, these MMP polymorphisms may confer an increased risk for adverse cardiovascular remodeling at a much younger age. In a robust sample size of hypertensive patients (>42,000), Tanner and colleagues identified interactions between antihypertensive agents to that of MMP-9 and MMP-12 polymorphisms and subsequent cardiovascular events. This suggests that identifying specific MMP polymorphisms, particularly in more vulnerable patient populations, may provide additional guidance for specific pharmacotherapy. For example, MMP-1 polymorphisms have been shown to provide additive prognostic value in patients with acute coronary syndromes, and thus may identify a subset of patients for more intensive pharmacotherapy. Of course, simple sequence substitutions within the MMP gene or the promoter region provide only a partial picture into the complex regulation of MMPs within the cardiovascular system. Nevertheless, there is growing evidence that polymorphisms that affect MMP transcriptional control may play a critical role in how these proteases contribute to cardiovascular remodeling and, ultimately, cardiovascular outcomes.
Matrix Metalloproteinase Posttranscriptional Regulation
Posttranscriptional events are directly influenced by small noncoding RNA molecules ( see also Chapter 2 ) termed microRNAs (miRs). miRs can bind to mRNA and interfere with the initiation of translation and/or accelerate mRNA degradation. As depicted in Fig. 4.3 , it is becoming apparent that specific miRs will cause posttranscriptional alterations in functional clusters of proteins that would be relevant to LV remodeling and to the ECM in particular. Largely utilizing mouse models of MI and aortic constriction, preclinical studies have investigated the effects of specific miRs, as well as the associated mechanisms on ECM remodeling and myocardial fibrosis. For example, miR-328 was found to upregulate TGF-beta and increase fibrillary collagen deposition. Other miRs, such as miR-19b, advance myocardial fibrosis by promoting fibroblast proliferation and migration. Furthermore, miR-21 and miR-155 are among a subset of miRs that contribute to fibrosis by both increasing profibrotic growth factors and promoting fibroblast survival. Conversely, a robust group of miRs, such as miR-29, miR-214, or miR-133, have been associated with antifibrotic outcomes. However, there are not always clear categorical distinctions between pro- and antifibrotic miRs. For instance, miR-15 has been observed to influence all of the aforementioned pathways. As the complex relationships between miRs and LV remodeling have become better understood, the clinical potential of miRs in cardiovascular disease has become apparent as well.

Clinical studies are demonstrating the prognostic potential of these miRs to identify those patients at increased risk for HF and to diagnose HF and monitor disease progression. miRs can be actively transported into the interstitial space, and thus, a pool of miRs exist within the ECM. These miRs can egress to the systemic circulation allowing for detection in patient plasma. For example, miR-34a promotes fibroblast survival, and plasma levels can predict patient development of ischemic HF after an MI. In patients with chronic HFrEF and HFpEF, the temporal trends of miR-22 correlated with the composite primary outcome of HF hospitalization, cardiovascular mortality, cardiac transplant, or LV assist device placement. Additionally, miR concentrations in the plasma of patients with various etiologies of HF have been screened against those in healthy controls to detect miR patterns unique to HF. From these studies, miR-21 was identified as being consistently upregulated in the plasma of HFrEF patients and can make a diagnosis of HFrEF with high sensitivity and specificity. miR-21, along with miR-208 and miR-1, also correlate with measurements of disease severity and may aid in identification of disease progression. These trends are further depicted graphically in Fig. 4.4 .

Similar screening studies have compared plasma miRs in patients with HFrEF to those in patients with HFpEF. Beber et al. reported that co-testing of five miRs present in the plasma could distinguish between HFrEF and HFpEF. Included in this group of miRs are three that are known to regulate ECM remodeling: miR-30, miR-146, and miR-328. Patterns in HFrEF and HFpEF of these and additional miRs involved in ECM remodeling are presented in Table 4.2 . The discovery that miRs can influence ECM remodeling in patients with HFrEF and HFpEF has led to efforts to harness the potential of miR expression as a therapeutic. This concept has been investigated in mouse models of aortic constriction and hypertension. Overexpression of the antifibrotic miR-29 decreased LV fibrosis, and reduced expression of profibrotic mediators such as TGF beta. miR-214 has also been implicated as a potential therapeutic as infusion in angiotensin II treated mice attenuated cardiac fibrosis and decreased the expression of TGF beta and fibrillary collagen in mouse models of acute MI. Additionally, transgenic overexpression of miR-133 in mice undergoing transverse aortic constriction improved myocardial fibrosis as well as diastolic dysfunction. Translating these concepts from animal models to clinical research has the potential to introduce a novel strategy for treating both HFrEF and HFpEF.
miR | Effect | Pattern in HFrEF | Pattern in HFpEF | Citations |
---|---|---|---|---|
miR-21 | Fibroblast survival and growth, ECM deposition | Increased, correlated with disease severity | Increased | 46, 52, 53, 55, 57–60, 152, 153, 177 |
miR-155 | Fibroblast proliferation and inflammation, collagen deposition | Increased | ? | 61, 72 |
miR-208 | Regulate collagen deposition | Increased in end stage HFrEF | ? | 29, 30, 56, 75 |
miR-133 | Reduce collagen expression and cardiomyocytes apoptosis | Decreased after cardiac injury, increased in end stage HFrEF | ? | 56 |
miR-30 | Decrease collagen deposition and regulate apoptosis | Decreased | Decreased | 31, 63, 76 |
miR-328 | Regulate profibrotic pathways | Decreased | Decreased | 76 |
miR-146 | Regulate collagen deposition | Decreased | Decreased | 27, 28, 76 |
miR-1 | Reduce ECM deposition | Decreased, correlated with disease severity | ? | 70, 71, 153 |
miR-150 | Inhibit activation of cardiac fibroblasts | Decreased in end stage HFrEF | ? | 73 |
Matrix Metalloproteinase Posttranslational Regulation
While the level of MMP synthesis is an important determinant of matrix degradation, the true degradative capacity of the MMPs is dependent upon the activation of the MMP pro-enzyme. After being synthesized in a latent, pro-enzyme, or zymogen form, the MMPs are secreted into the extracellular space. In order for MMP zymogen activation to occur, a sequence of proteolytic events must take place. Thus, an important control point or MMP activity is proteolytic cleavage by other enzymes (e.g., serine proteases and other MMP species), or by exposure to certain physical and chemical effectors. It has been demonstrated that serine proteases, such as plasmin, can generate active MMPs. In addition, MMP activation can also occur at the cell surface involving the MT-MMPs. At the cell membrane, MT-MMP is already in an active state, so it can then activate other MMP species at the cell surface. It has been clearly established that MT1-MMP activates MMP-2, and in fact may be the predominant pathway for MMP-2 activation. Moreover, it appears that a very wide proteolytic portfolio for MT1-MMP exists, which would imply that this MMP type may participate in a number of enzymatic and signaling cascades within the myocardial ECM. This is likely to hold particular relevance since robust levels of MT1-MMP have been identified within both experimental and clinical forms of HF.
Endogenous Matrix Metalloproteinase Inhibition
Another important control point of MMP activity is in the inhibition of the activated enzyme by the action of a group of specific MMP inhibitors, termed tissue inhibitors of matrix metalloproteinases (TIMPs). There are four known TIMP species of which TIMP-1 and TIMP-2 are the best characterized. The TIMPs are low-molecular-weight proteins that can complex noncovalently with high efficiency to MMPs in a 1:1 molar ratio. Therefore, these inhibitory proteins are an important endogenous system for regulating MMP activity in vivo. While the TIMPs are expressed in a variety of cells, TIMP-4 shows a high level of expression in cardiovascular tissue. While a predominant characteristic of the TIMPs is in the inhibition of active enzymes, this is likely to be an overly simplistic view of the function of these proteins. For example, studies have demonstrated TIMPs to be involved in the process of MMP activation, to exhibit growth factor–like properties, and to participate in apoptosis. For example, the over-expression of TIMP-1, -2, -3, -4 was induced in murine myocardial fibroblasts by adenoviral mediated transduction. In this study, a number of differential biological effects were observed by TIMP induction, which included the effects on collagen synthesis and apoptosis. These effects were not due to MMP inhibition since pharmacological MMP inhibition did not recapitulate these effects; therefore this indicated a direct action of TIMPs on fibroblast function. Moreover, TIMP-specific effects were observed in which TIMP-2 caused a robust increase in collagen synthesis, whereas TIMP-3 accelerated fibroblast apoptosis. Using transgenic constructs, differential effects of TIMP-3 have been observed in terms of LV remodeling and progression to HF, whether due to MI or pressure overload. More recent studies have identified that delivering a recombinant TIMP-3 post-MI can reduce adverse ECM remodeling and the progression of LV failure. These results underscore the highly diverse and complex nature of these TIMPs that exists within the myocardial interstitium and not only holds potential diagnostic/prognostic potential but also therapeutic relevance.
Heart Failure With Reduced Ejection Fraction and Extracellular Matrix Remodeling—Myocardial Infarction ( see also Chapter 34 , Chapter 19 )
A prolonged ischemic event, with or without reperfusion, causes a cascade of biological events that can be considered a wound-healing process and thus the canonical phases of wound healing are often utilized, which consist of three overlapping phases: inflammation, proliferation, and maturation. The first phase is the acute period in which reactive oxygen species, bioactive signaling molecules, and peptides released from the local environment cause inflammatory cell recruitment and invasion. The initiation of the inflammatory phase is likely due to the release of molecules as a result of myocyte death, and in turn induce the expression of cytokines, such as TNF alpha and the interleukins. A more comprehensive examination of cell viability and inflammation are found elsewhere in this text. Some key aspects of the early post-MI inflammatory process include: (a) activation of the intracellular transduction pathway, nuclear factor kappa B; (b) induction of a diverse family of chemokines; and (c) neutrophil recruitment and margination. While initially considered a rather monochromatic cell type in terms of form and function, the macrophages within the MI region appear to be quite heterogeneous and may ultimately influence the magnitude and direction of the localized inflammatory process. While this inflammatory process is critical to initiate a reparative response following myocardial injury, it appears that unlike other sites of tissue injury, the inflammatory response is prolonged within the MI region. The second phase is heralded by proliferation and transdifferentiation of myocardial fibroblasts into myofibroblasts. Further, released matrix structural proteins, such as fibrillar collagens, and matrix–integrin–cytoskeletal interactions facilitate contraction of the wound. The third phase of the wound-healing process normally results in complete contraction of the wound, apoptosis of the myofibroblasts, and the formation of a relatively acellular scar. However, in the context of post-MI remodeling, this canonical set of events does not necessarily occur. Rather, there is a continued proliferation and transdifferentiation of fibroblasts within the MI and border regions accompanied by a shift in matrix proliferative/degradative pathways within these transformed cells.
Significant changes within the ECM occur during all time points post-MI and likely contribute to the overall adverse LV remodeling process. First, the inflammatory response causes the release of MMPs as well as other proteases to degrade the ECM and allow for margination of inflammatory cells. However, with a persistent inflammatory state, MMP induction will also destabilize the newly formed ECM and the nascent scar. Second, the continued proliferation and altered expression of myofibroblasts within the MI region in terms of the balance between MMP and TIMP levels cause ECM instability and infarct expansion. Specifically, the transformed fibroblast population within the MI region likely results in a shift in the relative balance of MMPs and TIMPs, favoring accelerated ECM turnover and the failure of mature scar formation. Third, a robust expression of “profibrotic” signaling molecules occurs within the myofibroblasts and the residual myocyte populations, such as TGF, but this does not result in a well-formed ECM. Specifically, TGF signaling is enhanced within the MI region and causes significantly higher fibrillar collagen transcription; however, a number of critical posttranslational events involving collagen assembly are likely to be defective. For example, a loss of the matricellular protein, secreted protein acidic and rich in cysteine (SPARC), which is involved in procollagen processing to a mature collagen fibril, resulted in impaired collagen maturation and MI scar formation. Further, the interactions of the glycoproteins fibronectin and tenascin C may directly alter cell–ECM interactions, whereby other bioactive molecules, such as osteopontin, can directly influence ECM biosynthesis through a number of signaling pathways. Transmembrane receptors and co-receptors, such as endoglin and syndecan, which can modify profibrotic signaling through TGF and other growth factors, also likely play an important role not only in ECM synthesis and degradation but also in terms of myofibroblast transformation. Thus, abnormalities in fibroblast phenotype coupled with defects in both ECM synthesis and degradation pathways possibly converge to cause a feed-forward process in terms of MI wall thinning and dyskinesis, increased radial wall stress, and local strain patterns, and ultimately contribute to overall infarct expansion, LV remodeling, and HFrEF. These pathways and processes, as they may relate to the prognosis and diagnosis of HFrEF, are presented in a subsequent section.
Heart Failure With Reduced Ejection Fraction and Extracellular Matrix Remodeling-Dilated Cardiomyopathy ( see also Chapter 20 )
DCM constitutes a broad classification of primary myocardial disease states, which contribute to a significant proportion of patients with HFrEF, and several chapters within this text examine in detail the several animal models of DCM that have identified increased MMPs within the myocardium. In the rapid pacing DCM model, increased abundance of several MMP types occurred, with a loss of ECM structural integrity that coincided with the onset of LV remodeling and dilatation. Moreover, these changes in MMP myocardial levels and LV geometry preceded significant alterations in cardiomyocyte contractile function, which underscored the functional importance of MMP-mediated ECM disruption as an early event in this model of DCM. More definitive cause–effect relationships between LV myocardial remodeling and MMP induction have been established through the use of pharmacological MMP inhibitors. For example, MMP inhibition in the pacing DCM model attenuated the degree of LV dilation and dysfunction. In the canine model of ischemic DCM, pharmacological MMP inhibition attenuated the progression of LV dilation and systolic dysfunction. Thus, a contributory mechanism for the LV remodeling that occurs during the experimental form of DCM is MMP induction and heightened MMP activity within the LV myocardium.
A number of studies have examined relative MMP and TIMP expression in end-stage human DCM. Uniformly, these past studies have demonstrated an increase in certain MMP species in end-stage human HF. One of the first studies was by Gunja-Smith and colleagues in which increased myocardial MMP gelatinolytic activity was reported in DCM. Using immunoblotting procedures, subsequent studies identified that several MMP species were increased in LV myocardial extracts taken from patients with end-stage DCM. For example, increased MMP-3 and MMP-9 levels were observed in the DCM myocardial extracts. While MMP-13 was barely detected in the normal myocardium, a robust immunoreactive signal for MMP-13 was observed in DCM myocardium. Interestingly, MMP-13 has been associated with pathological remodeling states, such as breast carcinomas. The most robust change in MMP types within the DCM myocardium was that of MT1-MMP. A persistent induction and expression of MT1-MMP occurred in DCM fibroblasts when compared to myocardial fibroblasts harvested from nonfailing patients. Li et al. provided evidence to suggest that changes in the MMP/TIMP stoichiometric ratio occurred with end-stage DCM disease. In DCM, an absolute reduction in MMP-1/TIMP-1 complex formation has been observed. In a transgenic model of TIMP-3 deletion, a DCM phenotype was reported as a function of age. In DCM patients, chronic unloading of the LV through the use of ventricular assist devices was associated with a reduction in LV chamber dilation and myocardial MMP levels. Taken together, these results suggest that reduced MMP/TIMP complex formation may occur in cardiomyopathic disease states, which in turn would contribute to increased myocardial MMP activity and ECM remodeling.
Heart Failure With Reduced Ejection Fraction and Extracellular Matrix Remodeling—Hypertensive Heart Disease ( see also Chapter 25 )
The fundamental milestone in the development and progression of HFpEF is that of concentric LV hypertrophy, which is due to accelerated growth of both the myocyte and ECM compartments. The stimulus for LV hypertrophy is that of a prolonged pressure overload, which can arise as a function of hypertension, aortic stenosis, and arterial stiffening as a utility of age. While LV hypertrophy is initially an appropriate adaptive response, continued myocyte growth and expansion of the ECM will eventually lead to fundamental defects in LV diastolic performance. The physiology and pathophysiology of diastolic performance and clinical considerations of HFpEF are the subjects of a subsequent chapter. While a number of active and passive properties of the myocardium are altered in HFpEF, a predominant determinant is changes in ECM structure and content. Specifically, increased fibrillar collagen content as well as the accumulation of other nonstructural ECM proteins are invariable structural events in progressive LV hypertrophy and diastolic dysfunction. The continuous and potentially accelerated accumulation collagen within the ECM will reduce LV myocardial compliance and thereby impair the passive LV filling phase of diastole, and present as a resistance to flow with atrial contraction. The summation of these changes in both myocyte and ECM structure and function with progressive LV hypertrophy is a rise in LV filling pressures and, by extension, increased pulmonary capillary wedge pressure (PCWP). The increased PCWP will in turn contribute to higher pulmonary venous pressures and ultimately yield two of the more consistent and severe symptoms associated with HFpEF: exercise intolerance and pulmonary congestion. Finally, with persistently increased LV stiffness (both active and passive) and a higher PCWP, left atrial (LA) enlargement occurs, and thus measures of atrial size can also be indicative of worsening LV diastolic function and progression of HFpEF. Clinical studies have demonstrated that both direct and indirect measurements of ECM remodeling, particularly myocardial collagen accumulation, are directly related to increased PCWP as well as LA volumes.
As shown in Fig. 4.2 , key changes in ECM structure and function include fibroblast proliferation/transdifferentiation as well as fibrillar collagen accumulation. In pressure overload secondary to aortic stenosis, the significant myocardial collagen accumulation was associated with a concomitant increase in relative myocardial TIMP levels. Experimental studies support the concept that diminished myocardial MMP activity can facilitate collagen accumulation in developing hypertrophy. In the spontaneously hypertensive rat, the development of compensated hypertrophy is associated with increased myocardial TIMP levels, which would imply reduced MMP activity. The changes in myocardial MMP activation is likely to be time dependent and may not be constant throughout the development of pressure overload hypertrophy. Furthermore, the utilization of a microdialysis method to directly measure MMP interstitial activity demonstrated that MMP activity was reduced as a function of LV afterload. In a large animal model of progressive aortic stenosis that recapitulated features of HFpEF, changes were induced in MMP and TIMP profiles, which would favor ECM accumulation. In a murine model of LV pressure overload, genetic deletion of MMP-2 reduced the degree of myocardial fibrillar collagen accumulation and improved the indices of LV diastolic function. Since the primary mechanism for conversion of pro-MMP-2 to active MMP-2 is through complex formation with MT1-MMP, the induction of this MMP type may be pivotal in the changes in ECM structure and content that occur in HFpEF. MT1-MMP expression is sensitive to changes in mechanical load, whereby increased wall tension proliferated MT1-MMP promoter activity in vitro. Increased myocardial MT1-MMP expression has been identified in patients with LV pressure overload. In animal models of LV pressure overload, an early and sustained induction of MT1-MMP has been identified. For example, increased MT1-MMP promoter activity, and subsequently MT1-MMP proteolytic activity, has been reported following the induction of LV pressure overload in mice. The pathways by which MT1-MMP contributes to adverse ECM remodeling likely include facilitating proteolysis of interstitial molecules directly (such as integrins), amplification of active MMP-2 causing ECM instability, and abnormal architecture, as well as through enhancing profibrotic signaling pathways. Specifically, an important proteolytic relationship possibly exists between MT1-MMP and the subsequent activation of the profibrotic signaling molecule TGF, which would hold particular relevance in the context of LV pressure overload. Competent signaling of TGF requires release from a latency-bound state, binding to the TGF receptor complex, and induction of the transduction elements-Smads. TGF activation through Smads not only will induce fibrillar collagen expression, but also will facilitate fibroblast transformation/transdifferentiation. Thus, a likely important proteolytic interaction exists between certain MMP types, such as MT1-MMP, and the TGF signaling axis in terms of ECM accumulation and the progression to HFpEF.
While certain MMP types, such as MMP-2 and MT1-MMP, appear to be uniformly increased with LV pressure overload, other MMP types, such as the interstitial collagenase MMP-13, have been reported to be decreased. Other MMP types, such as MMP-1 and MMP-3, appear to be either unchanged or reduced with LV pressure overload. Interestingly, transgenic expression of human MMP-1 in mice (this MMP type is absent in rodents) and induction of LV pressure overload resulted in a relative reduction in myocardial fibrillar collagen content and improved indices of LV function. These findings suggest that the loss of normal constitutive levels of certain MMP types, or failure of an induction of certain MMP types with LV pressure overload, may facilitate abnormal ECM accumulation and adverse myocardial remodeling. This process does not occur in isolation, and key matricellular proteins and signaling molecules, such as thrombospondin and osteopontin, are also likely to play a key role in abnormal ECM composition with LV pressure overload and progression to HFpEF. These determinants of ECM regulation with HFpEF are examined further in the context of diagnostic/prognostic potential.
Myocardial Extracellular Matrix Remodeling in Heart Failure—Diagnostic Potential
Chapter 33 has been dedicated to biomarker profiling and HF, and thus the focus herein will be to examine specific biomarkers that hold relevance to ECM remodeling, with particular attention to those that hold diagnostic/prognostic potential. In terms of plasma biomarker profiling with HFrEF and a post-MI etiology, plasma troponin levels and other biomarkers are obviously relevant; thus it is likely that a specific portfolio of ECM biomarkers will provide specific prognostic information in the post-MI period. For example, past studies have demonstrated that increased plasma levels of collagen peptide fragments occur following MI and in progressive HFrEF. In keeping with ECM degradation, the induction of ECM proteases, such as the MMPs, occur in patients following MI and likely hold predictive value in terms of identifying those patients at greatest risk for the early development of HFrEF. For example, the magnitude of plasma MMP-9 levels that occur within the early post-MI period is associated with a greater degree of LV dilation at 6 months post-MI. In a study by Wagner and colleagues, the early increase in MMP-9 levels was associated with an increased risk of the later development of HF (odds ratio of 6.5). In a recent report that compiled the results from approximately 60 studies in patients post-MI, those that were most consistently present in prediction models included indices of neurohormonal activation, such as brain natriuretic peptide and indices of ECM degradation, such as MMP-9. Cytokine/chemokine release that would cause the induction of pathways relevant to ECM degradation and fibroblast transformation, as well as the release of matrikines, all occur in patients in the post-MI period. Other matrix-related molecules, such as galectin-3, have been associated with determinants of ECM remodeling but are not necessarily independently predictive of HFrEF. In addition to plasma biomarkers for ECM degradation, increased markers for ECM synthesis have also been identified, which include the TGF co-receptor endoglin and the matrikines tenascin-C, osteopontin, and syndecan-4. In large survey studies that included subjects with MI and DCM, plasma levels of MMPs and TIMPs have provided clear prognostic information with respect to cardiovascular events and mortality. Finally, plasma profiling of these determinants of ECM remodeling may hold value as both a diagnostic and a therapeutic predictor, that is, theragnostic. For example, retrospective plasma samples taken from HFrEF patients with a 12-month follow-up after cardiac resynchronization therapy (CRT) identified that TIMP-1 levels may hold predictive value in terms of CRT response.
Since the ECM likely plays a critical role in the pathophysiology of HFpEF, strategies that can evaluate and quantify changes in this entity hold prognostic relevance. A summary of representative plasma biomarkers that have been evaluated in the context of HFpEF that may hold relevance to ECM remodeling is presented in Table 4.3 . For example, plasma profiles of peptide fragments of fibrillar collagens (types I and III) have been used in patients with developing and established HFpEF. The collagen fibril may undergo posttranslational modification by enzyme mediated cross-link reactions as well as nonenzyme mediated cross-link formation. Of note, nonenzymatic cross-link formation can be mediated by advanced glycation end products (AGEs). AGEs bind to specific cell membrane receptors that include the receptor for AGE (RAGE). Since increased fibrillar collagen stability can occur through increased RAGE interactions, profiling plasma levels of soluble RAGE (sRAGE) as well as indices of AGE formation may hold prognostic/functional relevance in HFpEF. A biologically relevant consequence of increased collagen AGEs is reduced susceptibility to MMP-mediated degradation and turnover. Significant and directional changes in the plasma profiles of MMPs and TIMPs occur in patients with developing HFpEF and have been shown to hold potential prognostic utility. For example, a specific cassette of MMPs and TIMPs, coupled with N-terminal pro b-type natriuretic peptide (NT-BNP), provided significant prediction in terms of patients at risk for the development of HFpEF. Other ECM chemokines/matrikines, such as cardiotrophin, have been associated with the development and progression of HFpEF. It is notable that the recent American Heart Association scientific position statement regarding the use of biomarkers for HFpEF identified a number of ECM-related proteins and pathways, which are those prominently identified in Table 4.3 .
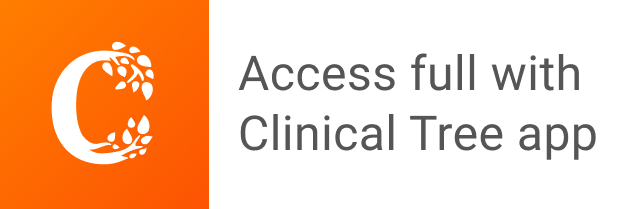