Keywords
Heart-functional morphologyRotational vortexIrrotational vortexLemniscatesMyocardial fibersMyocardial twistWiggers diagramVentricular myocardial bandOpposing force modelIntracardiac flow patternsHelical aortic flowFlow-restraining functionThe primary function of muscles is the generation of force or movement in response to physiological stimulus. The myocardium is a heterogeneous mass of muscle tissue which occupies a unique transition between the involuntary (smooth) and the voluntary (striated) muscles. While the individual motor units in a voluntary muscle are supplied by the nerve endings and can be recruited at will across the spectrum of forces, the cardiomyocytes are electrically connected and function as a single unit, a syncytium that contracts in unison with each impulse autonomously generated by a specialized group of pacemaker cells within the heart itself. Unlike the skeletal muscle and its fibers, the myocardium has neither origin nor insertion. Rather, the defining structural component of the heart is the arrangement of myocytes within the supportive connecting tissue matrix, to form higher order aggregates. Despite hundreds of years of anatomical dissection, many details of the heart’s structure, as related to its function, remain unresolved. In particular, the current models applied to the understanding of heart failure remain insufficient in the face of the repeated attempts to develop a unifying hypothesis [1]. To address this concerning trend, the US Institute of Health organized a symposium in 2002 entitled “Form and Function: New Views on Development, Diseases and Therapies for the Heart,” with the objective to deepen the understanding of the ventricular structure and function in light of basic and clinical sciences and to identify new insights from functional geometry and their effective integration into therapies [2]. In addition to taking stock of the existing knowledge in light of the numerous in vivo imaging modalities developed over the past decades, the symposium spurred a wave of multidisciplinary research on the basic structure and function of the heart and ignited an ongoing debate.
13.1 Historical Perspective
When the longitudinal fibers of the heart contract, while all other relax and distend, the heart appears shorter but larger: this presents dilatation (diastellomene; diastole). The opposite occurs when the longitudinal fibers relax but the transverse fibers shorten and so the heart becomes contracted (systellomene; systole). [5]
Thus, for Galen, both systole and diastole are active processes brought about by different sets of muscles. Diastole clearly played the superior role because the dilating ventricles “attracted” blood by suction, much like the blacksmith’s bellows or a loadstone, contributing to forward flow of the blood. This view was refuted by Harvey who regarded systole as active and diastole as a purely passive process (cf. Sect. 22.1).
It seems to me that when the heart exerts a full power of attraction, it would actually tear a vessel into pieces if our Creator had not in this instance too contrived a protection against such an accident by placing outside each opening that admits material another separate cavity like a storehouse for nutrient, so that the vessel may not be in danger of rupturing when at times the heart attracts suddenly and violently and the vessel alone, because it is narrow, cannot furnish abundantly all that the heart demands. (quoted in [6])
Resemblance between helical forms and flows in nature and the myocardial structure was also known to the Renaissance anatomists and has been a subject of fascination to researchers ever since. Leonardo da Vinci believed that the heart is a “vessel made of dense muscle” and proposed that closure of the aortic valves results from vortical flow in the sinuses of Valsalva [7]. Leonardo may have been the first to describe the difference between the rotational and irrotational vortex, a key concept of circulation in hydrodynamics [8].1 Leonardo was also the first to observe shortening of the long axis of the heart during systole [9]. Richard Lower published the first detailed drawings of the separate myocardial muscular layers—overlapping much like rings of an onion—and drew attention to the fact that the endocardium and epicardium meet directly at the apex which is free of muscular elements [10]. In 1728, Senac demonstrated that the myocardial fibers are organized in spiral, three-dimensional arrangement, a finding repeatedly confirmed by investigators over the next 200 years (for review see [11–13]).
Among the numerous available studies of anatomical dissections of the heart, the morphological descriptions and superb illustrations by Scottish anatomist J. Bell Pettigrew stand out and continue to be quoted as a classic in the field [3]. In his monumental work “Design in Nature” published in1908, Pettigrew draw attention to the ubiquitous presence of spiral forms in all kingdoms of nature and suggested that understanding of the common architectural plan of “perplexing” arrangement of myocardial fibers is a “Gordian knot” of anatomy holding the key to its function [14]. Pettigrew maintained that the heart is a transformed blood vessel where the thin-walled atria represent the continuation of the great veins, while the thick-walled ventricles and aortic and pulmonary outflow tracts mark the beginning of the arteries. He further argued that, unlike voluntary muscles, myocardial fibers have neither origin nor insertion, but form continuous spirals (lemniscates) between the apex and the base and, moreover, exhibit spontaneous rhythmical movements of the syncytium.
The spiraling and looping pattern of myocardial fibers, including the vortex cordis, is a reflection of intracardiac blood movements. In the heart, form and movement unite in a rhythmical process in space and time: the organ, a form in space, is simultaneously a movement in time…the movement of the cardiac walls is a physical replication of the creative fluid flow movements which they enclose. [16]

Posterior view of the left ventricle of a sheep’s heart with successive layers of the myocardium removed, as dissected and photographed by Pettigrew-Bell in 1864. Note the nearly vertical course of the outer and the innermost layers running in the opposite directions and a horizontally orientation of the mid-wall fibers. (Reproduced from ref. [14])

Schematic representation of left ventricular architecture in three dimensions (a). Superficial fibers originate at the base and sweep longitudinally toward the apex where, turning sharply, they continue as the middle (horizontal), or inner, vertical layer forming the papillary muscles or return to the base. (b) A stylized drawing of the vortical structure of the heart, demonstrating deep and superficial helical fibers running in the opposite direction. The outer layer has been “peeled” and inverted to show the counter-directional course of the inner and outer layers. (c) Schematic representation and (d) interlocking spirals at the heart’s apex. ((b) adapted from ref. [17]; (c) and (d) adapted from ref. [14])
Other, perhaps less phenomenologically oriented, researchers have found it difficult to reproduce Pettigrew’s ingenious dissections and morphological descriptions of discrete muscular layers within the ventricular walls. The preconceived notion that the heart is an organ of blood propulsion has compelled some to emphasize the elements in myocardial architecture which would primarily make it into an efficient pump. (Reviewed by Brecher and Galletti [18].) Carl Ludwig introduced the concept of a muscular cylinder as the principal structure of the left ventricle enveloped by endocardial and epicardial layers crossing at the right angles of its longitudinal axis in the form of an X [19].This model was further refined by von Krehl who named the circular fibers “Triebwerkzeug,” or “propelling tool,” in literal translation from the German [20], technically known as the “actuating fibers.” The concept gained further momentum with the work of MacCallum who stressed the crucial role of the cardiac “fibrous skeleton” [21]. According to MacCallum’s anatomical model, four interconnected fibrous rings at the orifices of the great vessels and the AV orifices serve as attachments of cardiac valves, and also as points of insertion for ventricular muscles, of which several groups had been identified by functional anatomists at the time, such as the “bulbo-spiral” and “sino-spiral” tracts described by Mall [22]. It should be noted parenthetically that during the time, a functional similarity between the skeletal muscle and the myocardium was emphatically supported by Frank [23] and Starling [24, 25] and that seminal experiments were performed on the isolated heart preparations culminating in Starling’s formulation of the “law of the heart” [26] (see Sect. 16.1). Subsequent work demonstrated that in comparison to the skeletal muscle, the myocardium is capable of developing only a fraction of active tension (2–5 kg/cm2 vs. 200 g/cm2 of cross section of the muscle) [27]. Frank’s interpretation of myocardial dynamics nevertheless became universally accepted [28, 29].

A composite diagram of angles (yellow lines) followed by myocardial fibers at different depths relative to the equator of the left ventricle, as demonstrated by Streeter and coworkers. (a) The circular or actuating fibers, given prominence by Krehl (1881) were thought to play a pivotal role in the pressure-propulsion model of the heart. (Reproduced from ref. [34], used with permission of Oxford University Press). (b) Schematic cross-section of the ventricles showing relative thickness of wall components. Thin apical segment denotes a poorly designed pressure-propulsion pump. RV right ventricle, S septum, LV left ventricle

A section of ventricular wall at low magnification showing a uniform orientation of individual myocytes. The spaces between the cells (yellow arrows) are filled by the supporting fibrous matrix (a). A scanning electron micrograph of endomysial weave, surrounding each individual myocyte, and a pair of coiled perimysial fibers that bind groups of myocytes into so-called myofibers (b). Three-dimensional architecture of the supporting myocardial matrix suggests that, in comparison with skeletal muscle, the myocardium is well protected to withstand excessive filling (distension) (c). (Reproduced from ref. [34], used with permission of Oxford University Press)

Diffusion tensor magnetic resonance imaging (DTMRI) of the three-dimensional track progression of myocardial fibers of the base (a), apex (b), papillary muscles (c), and mid-wall section (d) of the myocardium. Individual tracks are color coded and extend in a figure-of-eight pattern between the base and the apex of the heart. (Reproduced from ref. [36], used with permission of John Wiley and Sons)

(a) Whole rabbit heart volume rendering derived from micro-CT data, showing region of interest presented in panel b (white box). (b) Volume rendering of sub-endocardial tissue block derived from dataset presented in A, showing the 3D morphology of multiple lamellar units. (c) Single lamellar unit (orange) segmented from micro-CT data of the rabbit left ventricular posterior wall, with individual myocyte chains resolved. (Reproduced from ref. [37] used with permission of John Wiley & Sons)
13.2 Models of Ventricular Structure and Function

Myocardial contraction and twist during the cardiac cycle. During systole (a) the ventricles contract (red), the atrioventricular valves close, the semilunar valves open, and the valve plane is pulled toward the apex. The base of the heart rotates clockwise and the apex in the counterclockwise direction. In diastole (b), the atria contract (red) and the valve plane is pulled toward the atria. Torsional recoil in diastole occurs during isovolumic relaxation and early diastolic filling. (Adapted from [39] used by kind permission of Prof. J. W. Rohen)

Wiggers diagram of cardiac cycle events as interpreted by the conventional and ventricular myocardial band (VMB) models. In the conventional model the systole comprises of left ventricular isovolumetric contraction and ejection, enacted by concentric contraction of ventricular cone. In VMB model, the systolic ejection is produced by shortening of the ventricular cone with descent and torsion of the base (cf. Fig. 13.9). Note that active diastolic phase begins after peak ejection and corresponds to rapid ventricular filling marked by a dip in the pressure recording below the zero line (red arrow with asterisk). Similar sequence of events based on different anatomic interpretation of cardiac structure would apply to opposing force model (see text for explanation)
To address this deficiency, two additional but contrasting models have evolved over the past several years among the basic scientists and clinicians seeking to explain the movements of ventricular walls in the light of existing research. The first is the ventricular myocardial band (VMB) conceived by Francisco Torrent-Guasp, and the second, the “antagonistic force” model developed by Paul Lunkenheimer (see below).
13.2.1 Ventricular Myocardial Band
The concept of VMB introduced by Torrent-Guasp presents a significant deviation from the classical model presented above and a notable attempt to portray the heart not only as a propulsion, but as a “pressure-suction pump” [11, 40]. According to its adherents, the model optimally explains the mechanics of sequential myocardial contraction, including torsion and untwisting, and presents a “unifying approach to causative architectural mechanisms affecting the heart” [41]. It arose on the premise that the limited shortening capacity of myocardial sarcomeres (in the range of 10–20%) is inadequate to account for ventricular ejection fraction of up to 60%. Similarly, the thickening of left ventricular wall of up to 30% can only be explained with additional systolic “augmentation” [11]. Finally, rapid ventricular filling at the end of diastole, frequently accompanied by negative pressure (i.e. suction) cannot be explained solely by elastic diastolic recoil of the ventricle. To affect these changes, according to Torrent-Guasp and collaborators, participation of an additional contractile force is needed. This is as afforded by the VMB.

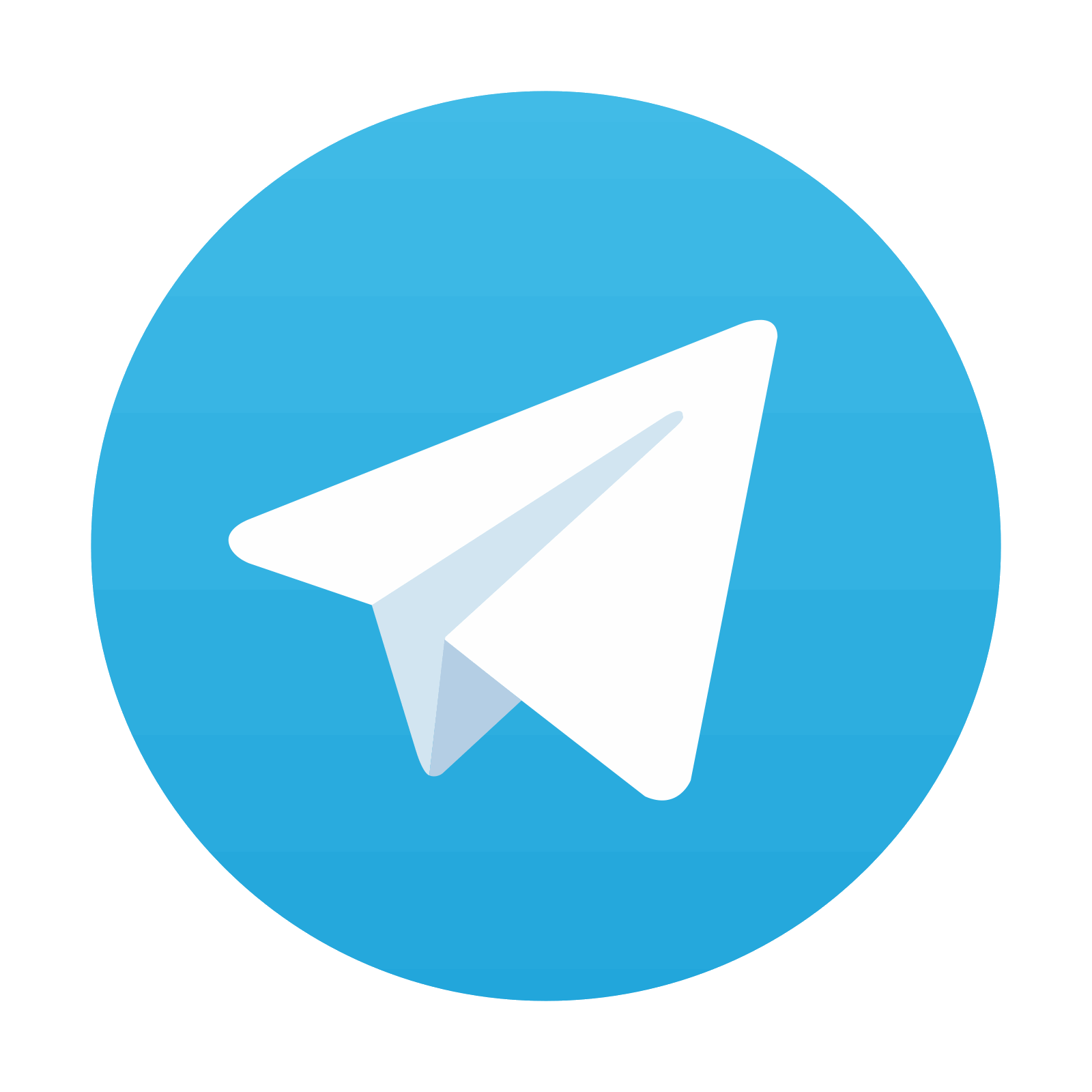
Stay updated, free articles. Join our Telegram channel
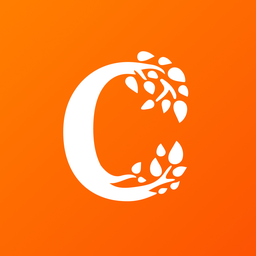
Full access? Get Clinical Tree
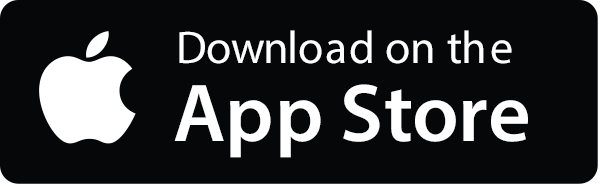
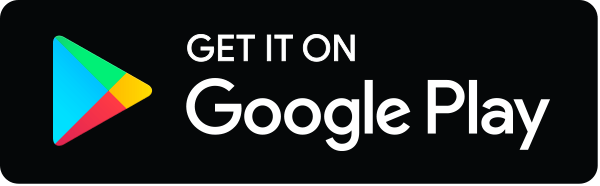