Outline
A Perspective on the Renin Angiotensin Aldosterone System, 76
The Biochemical Physiology of the Renin Angiotensin System, 76
Renin and Pro-Renin, 79
Other Nonrenin Enzymes, 79
Angiotensinogen, 79
Angiotensin I and II Forming Enzymes, 79
Angiotensin-Converting Enzyme, 79
Chymase, 80
Angiotensin-Converting Enzyme 2, 80
Other Angiotensin-Forming Enzymes, 80
Main Angiotensin Products, 81
Angiotensin-(1-12), 81
Angiotensin-(1-9), 81
Angiotensin I and Angiotensin II, 81
Angiotensin III and Angiotensin IV, 81
Angiotensin-(1-7), 82
Ala 1 -Angiotensin II and Almandine, 82
Angiotensin II Mediated Signaling Pathways, 82
Angiotensin II Receptors, 82
Ang II/AT 1 -R-Mediated G Protein-Coupled Pathways, 83
Ang II/AT 1 -R-Mediated Classic G Protein-Coupled Signaling Pathways, 83
Ang II/AT 1 -R-Mediated Transactivation of Receptor Tyrosine Kinases, 83
Ang II/AT 1 -R-Mediated Nonreceptor Tyrosine Kinases Activation, 84
Ang II/AT 1 -R-Mediated Mitogen-Activated Protein Kinases Activation, 86
Ang II/AT 1 -R-Mediated Reactive Oxygen Species Signaling, 86
G-Protein-Independent AT 1 -R Signaling Pathways, 87
Ang II/AT 2 -R-Mediated Signaling Pathways, 87
Summary and Conclusions, 88
A Perspective on the Renin Angiotensin Aldosterone System
Although Bright (1827) first associated kidney hardness with a strong arterial pulse, current knowledge regarding the role of the renin angiotensin aldosterone system to homeostasis has been gained over the last 50 years. It is not to be denied that the discoveries associated with characterization of this critically important circulating and tissue-borne hormonal system transcended the field of cardiovascular medicine, having a major impact on the research and treatment of renal, neurological, hepatic, and skeletal diseases. The broad role of the hormone angiotensin II (Ang II) and its biologically related main peptides—angiotensin-(1-7) (Ang-[1-7]), angiotensin III (Ang III), and angiotensin-(1-9) (Ang-[1-9])—in modulating immune and inflammation responses is a reason why drugs acting to inhibit, or block renin-angiotensin system effectors are showing benefits above and beyond their original indication. Antitumoral actions are documented for drugs preventing Ang II actions or increasing Ang-(1-7) activity. The ubiquitous mechanisms accounting for such a diverse spectrum of actions involve multifactorial signaling pathways.
This chapter reviews the molecular mechanisms of the renin-angiotensin system, and underscores a major opportunity to develop a new generation of drugs with an increased capacity to reach the intracellular sites at which locally borne angiotensins modulate organ function. The clinical applications of antagonizing the renin-angiotensin system are reviewed in Chapter 37 . For the convenience of the reviewer, a summary of the abbreviations used is presented at the end of this chapter.
The Biochemical Physiology of the Renin Angiotensin System
Initial studies of Ang II formation and action were influenced by the prevailing assumption that this peptide acted as a circulating hormone originating from the action of renal renin on the angiotensinogen (AGT) released from the liver. During the decades preceding the current millennium, enzymological studies were driven to answer how renal renin acted on the rate limiting step protein substrate that accounted for the generation of angiotensin peptides. Over a span of probably two decades, the accepted view of the system was that renal renin generated angiotensin I (Ang I) by splitting the bond between leucine 10 (Leu 10 ) and leucine 11 (Leu 11 ) from the liver derived AGT substrate. The early conclusions were partly misleading, as they failed to realize that valine 11 (Val 11 ) is substituted for Leu 11 in the human AGT molecule. Circulating Ang I was then further processed into Ang II by the action of angiotensin-converting enzyme (ACE), which hydrolyzed the bond between phenylalanine 8 (Phe 8 ) and histidine 9 (His 9 ) in the C-terminus of the Ang I molecule. The resulting molecule was Ang II, the main bioactive peptide of the renin angiotensin system. This conceptual understanding of the biochemical pathways for Ang II production implies the existence of a linear system in which the hydrolytic activity of renin and ACE sequentially cleaved Ang I from AGT and Ang II from Ang I. The 1980s began to force a reconsideration of these concepts as identification of Ang-(1-7) in Ferrario’s research program, and the heptapeptide fragment angiotensin IV (Ang IV) challenged the prevailing simplistic understanding of Ang II singularity in the regulation of blood pressure and fluid balances ( Fig. 5.1 ). A diagram of the family of angiotensin peptides derived from the N-terminal end of AGT is documented in Table 5.1 . Table 5.2 illustrates the location of the extended number of humans’ genes now recognized to account for the expression and function of the renin angiotensin system.

Peptide | Amino Acid Sequence |
---|---|
Angiotensinogen (AGT) | H 2 N-Asp 1 -Arg 2 -Val 3 -Tyr 4 -Ile 5 -His 6 -Pro 7 -Phe 8 -His 9 -Leu 10 -Val 11 -Ile 12 -His 13 -Asn 14 -Glu 15 -COOH |
Angiotensin–(1-12) | H 2 N-Asp 1 -Arg 2 -Val 3 -Tyr 4 -Ile 5 -His 6 -Pro 7 -Phe 8 -His 9 -Leu 10 -Val 11 -Ile 12 -COOH |
Angiotensin–(1-10) (Ang I) | H 2 N-Asp 1 -Arg 2 -Val 3 -Tyr 4 -Ile 5 -His 6 -Pro 7 -Phe 8 -His 9 -Leu 10 -COOH |
Angiotensin–(1-9) | H 2 N-Asp 1 -Arg 2 -Val 3 -Tyr 4 -Ile 5 -His 6 -Pro 7 -Phe 8 -His 9 -COOH |
Angiotensin–(1-8) (Ang II) | H 2 N-Asp 1 -Arg 2 -Val 3 -Tyr 4 -Ile 5 -His 6 -Pro 7 -Phe 8 -COOH |
Angiotensin A | H 2 N-Ala 1 -Arg 2 -Val 3 -Tyr 4 -Ile 5 -His 6 -Pro 7 -Phe-COOH 8 |
Angiotensin–(1-7) [Ang-(1-7)] | H 2 N-Asp 1 -Arg 2 -Val 3 -Tyr 4 -Ile 5 -His 6 -Pro 7 -COOH |
Almandine | H 2 N-Ala 1 -Arg 2 -Val 3 -Tyr 4 -Ile 5 -His 6 -Pro 7 -COOH |
Angiotensin–(1-5) | H 2 N-Asp 1 -Arg 2 -Val 3 -Tyr 4 -Ile 5 -COOH |
Angiotensin-(2-8) (Ang III) | H 2 N- Arg 2 -Val 3 -Tyr 4 -Ile 5 -His 6 -Pro 7 -Phe 8 -COOH |
Angiotensin–(3-8) (Ang IV) | H 2 N- Val 3 -Tyr 4 -Ile 5 -His 6 -Pro 7 -Phe 8 -COOH |
Gene | Chromosomal Location |
---|---|
Angiotensin-converting enzyme (ACE) | long (q) arm of chromosome 17 at position 23.3 |
Angiotensin-converting enzyme 2 (ACE2) | long (q) arm of chromosome X at position 22.2 |
Angiotensin II type 1 receptor (AGTR1) | long (q) arm of chromosome 3 at position 24 |
Angiotensin II type 2 receptor (AGTR2) | long (q) arm of chromosome X at position 23 |
Angiotensinogen (AGT) | long (q) arm of chromosome 1 at position 42.2 |
Carboxypeptidase A1 | long (q) arm of chromosome 7 at position 32.2 |
Chymase (CMA1) | long (q) arm of chromosome 14 at position 12 |
Elastase-2 (ELA2) | short arm (p) of chromosome 19 at position 13.3 |
Mas receptor (MAS1) | long (q) arm of chromosome 6 at position 25.3 |
MrgD Receptor | long (q) arm of chromosome 11 at position 13.3 |
Renin (REN) | long (q) arm of chromosome 1 at position 32.1 |
As illustrated in Table 5.1 , humans and rodents differ in the molecular sequence of the amino terminal end of the AGT molecule as valine replaces leucine in position 11. Additional significant differences exist in the amino acid sequence of human AGT beyond valine 11 (Val 11 ). Table 5.1 shows that the human amino acid chain beyond Val 11 is composed of Ile 12 -His 13 -Asn 14 -Glu 1 , while in the rat the amino acid sequence contains Tyr 12 -Tyr 13 -Ser 14 -Lys 15 . These amino acid differences influence the identity and affinity of the enzymes cleaving the biologically active angiotensins from AGT. Indeed, it has been well documented that rat renin has no hydrolytic activity on human AGT.
Contemporary research affirms the view that the renin angiotensin system is a complex system in which biological diversity is revealed not only by the different effects of the products generated from either Ang I or Ang II but also through its receptors and peptide-forming and degrading enzymes. That the renin angiotensin system was far more complex than generally accepted, and that this complexity guarantees a well-tuned adaptation of the blood supply of the body compartments to all physiological conditions, was advanced as early as 1991.
The principles of network biology allow a more precise understanding of the complex interactions between enzymes and products within the renin angiotensin system. The behavior of protein-protein and molecular interactions of the renin angiotensin system network is determined by the orchestrated activity of several components interacting with each other through pair-wise interactions. Our studies suggest the existence within the renin angiotensin system of four primary nodes for the deterministic control of hormone (Ang II and Ang-[1-7]) expression signaling cellular functions in terms of growth, contractility, protein synthesis, and gene expression. Fig. 5.2 shows the nodes at which enzymes catalyze reactions transforming one metabolite into another. The four nodes are represented as (a) Aogen processing enzymes; (b) neutral endopeptidases (NEPs; see also Chapter 9 ); (c) ACE; and (d) ACE2. At each node, a regulated process determines the secondary processing of a protein/peptide to a corresponding shorter fragment destined to serve as either a substrate for an additional processing at a secondary node or a final signaling action on the cell’s receptor. At the first node (Aogen processing enzymes), AGT, the sole known identified substrate for the generation of angiotensin peptides, is catalyzed into forming Ang I directly via circulating or tissue expressed renin. Within this same node, however, our recent studies show that AGT is a substrate generating another Ang I-forming precursor, the dodecapeptide angiotensin-(1-12) (Ang-[1-12]), by an enzyme that is not renin. The intermediate products upstream from Ang I (Ang I or Ang-[1-12]) now become substrates to be acted upon by either the neprilysin (NEPs node; see also Chapter 9 ) or the ACE node where these enzymes cleave Ang I to generate Ang II (ACE node) or transform Ang I into Ang-(1-7) (NEPs node). Importantly, the ACE node also functions to regulate the concentration of Ang-(1-7), since ACE degrades this heptapeptide into Ang-(1-5) (not shown). The fourth node (ACE2) cleaves Ang II into Ang-(1-7).

Establishing the characteristics of the factors regulating the activity of each node is not a trivial matter, as each component of the system can exert a regulatory influence on either the enzymes’ expression or activity and the product generated at each node. For example, Ang II plasma or tissue concentrations shows a feed forward interaction with the Ang I-forming enzyme node as the peptide stimulates the expression of AGT transcripts in the liver, heart, and kidneys. Interestingly, Ang-(1-7) modulates the activity of the ACE node by acting as a weak endogenous inhibitor of the C-terminal domain of the enzyme. In addition, Ang II exerts a negative regulatory interaction on ACE2 gene expression, a feedback interaction that is neutralized by Ang-(1-7).
Renin and Pro-Renin
The enzyme renin can be ubiquitously expressed by a variety of cells, as new evidence suggests its critical evolutionary involvement in tissue homeostasis only in part through its role in regulating body fluid volumes. Although renin is found in many different tissues, its highest expression in the juxtaglomerular cells of renal afferent arterioles constitutes the pivotal sensory mechanism that determines its release into the circulation. Importantly, other renal locations for renin synthesis and release are found in a subset of mesangial cells, arteriolar smooth muscle cells, interstitial pericytes, and distal nephrons and renal collecting duct. Studies reporting the existence of renin and prorenin in distal nephrons and collecting ducts substantiate the potential for renal tubular Ang II formation as an alternative mechanism that, independent of the blood pressure and sodium sensory mechanisms of the juxtaglomerular apparatus and the macula densa, could contribute to the pathogenesis of essential hypertension. . The active renin enzyme synthesized as preprorenin is converted into the active releasable form through loss of the signal peptide and removal of the 43-amino-acid pro-segment contained in prorenin. The discovery of a prorenin receptor (PPR) revealed a nonproteolytic mechanism for the activation of renin through the exposure of its catalytic site. Deletion of 10 bp in exon 5 of the Ren gene in the rat caused inactivation of the renin gene, loss of renin granules in juxtaglomerular cells, and significant alterations in renal morphology comparable to those obtained with renin cells ablation in mice.
It has not been possible to find sufficient discriminative renin activity by assay procedures in the circulation as a superior biomarker of an overactive renin angiotensin system. This failure contributed to the search for renin expression and action within the tissue environment, particularly within the vascular wall and the heart. It was assumed that renin present in the extracellular milieu of the heart and blood vessels could generate Ang I from the plasma substrate, leading to Ang II formation by the action of ACE contained by vascular endothelial cells or perivascular tissue. No definitive conclusion has been reached as to whether renin is synthesized by cells in tissues other than the kidneys or its presence in nonrenal tissues reflects a cellular uptake mechanism.
Renin release from juxtaglomerular cells is modulated by the renal baroreceptor through changes in renal perfusion pressure, the sensory of sodium (Na + ) by the macula densa, and renal sympathetic nerve activity. Additional regulation of active renin secretion is exerted by Ang II through renal AT 1 receptors, the primary intracellular second messenger cAMP, and arachidonic acid (AA) derivatives.
Other Nonrenin Enzymes
Over the years, several renin-like enzymes were postulated to release Ang I from either AGT or the model synthetic substrate tetradecapeptide angiotensin-(1-14) (Ang-[1-14]). Cathepsin D and cathepsin G, acid and neutral proteases, tonin, the serine protease Esterase B, kallikrein-like serine proteases, and mouse gamma-nerve growth factor (γ-NGF) are among the enzymes implicated as Ang l-forming enzymes. Tonin forms Ang II directly from a plasma protein, from the tetradecapeptide substrate Ang-(1-14), and from Ang I. Tonin is present in most tissues, but in highest concentration it is found in submaxillary glands. A neutral protease with Ang l-forming activity that could readily be separated from acid proteases and both plasma and renal renin was identified in the dog brain. Findings of extended forms of Ang I, Ang-(1-25), and the dodecapeptide angiotensin-(1-12) in human and rodents raise additional issues as to whether nonrenin mechanisms may be more critical than anticipated previously.
Angiotensinogen
This 452-amino acid protein is the only known forming precursor of angiotensin peptides. The liver is the primary site for its synthesis, although in more recent years extrahepatic sources of generation exist in visceral fat, renal tubules, brain, heart, and reproductive organs. The AGT protein is produced through the cleavage of a single gene pre-pro-AGT (see Table 5.1 ). Synthesis, processing, and glycosylation changes vary in relation to species and site of tissue generation. The levels of the AGT protein are influenced by glucocorticoids, estrogen, thyroid hormone, insulin, and Ang II. A positive feedback in which Ang II augments AGT production has been demonstrated in the kidney and the liver. This mechanism may act to prevent depletion of substrate during excess Ang II production. On the other hand, Ang II reduces AGT mRNA in adrenal glands. Genetic studies underscore the critical role for the substrate in contributing to hypertension pathogenesis. Studies in transgenic mice show that arterial pressure increases in proportion to the number of AGT gene copies. Likewise, deletion of the AGT gene is associated with lower resting blood pressure, while the Lu et al. recent study shows that hypo-expression of the gene in a low-density lipoprotein receptor −/− background is associated with reduced blood pressure, less atherosclerosis, decreased body weight gain, and liver steatosis. Emerging evidence regarding an interplay among radical oxygen species (ROS), inflammation, and Ang II is strengthened by the observation that oxidative stress exposes the cleavage site of the AGT molecule to renin. Single nucleotide polymorphisms (SNPs) in the AGT gene have been explored for hypertension linkage. Data suggest that T174 and M235T associates with hypertension. We showed that genetic variation of the M235T, but not the ACE (I/D), genotype contributes to the presence of coronary heart disease independently of blood pressure.
Angiotensin I and II Forming Enzymes
The past decade has forced a revision of accepted tenets regarding the enzymatic pathways forming Ang II and other biologically active renin angiotensin system peptides. The classic view that ACE (EC 3.4.15.1) is the critical enzymatic fulcrum for the generation of Ang II has been challenged by the finding that chymase (CMA1; EC 3.4.21.39) forms Ang II directly from Ang-(1-12). Neprilysin and thimet oligopeptidase (EC 3.4.24.15) cleave Ang I to produce Ang-(1-7) in the circulation, while renal neprilysin generates Ang-(1-7) from either locally contained Ang-(1-12) or Ang I. Less appreciated are potential roles of (a) carboxypeptidase A (EC 3.4.17) forming angiotensin-(1-9) from Ang-(1-12) or Ang-(1-7) from Ang II; and (b) the Ang II generating ability of elastase-2 (EC 3.4.21.37). The variety of alternate pathways for angiotensin peptide formation may explain the failure to sustain Ang II suppression in patients chronically dosed with effective amounts of ACE inhibitors. The importance of these alternate pathways, primarily at the tissue level, may explain why the actual quantitative reduction in cardiovascular endpoints obtained with this drug class is less than predicted on the basis of their mode of action and physiological actions.
Angiotensin-Converting Enzyme
Renin catalytic action on human AGT generates angiotensin I (Ang I) through the cleavage of the amino acid bond between leucine and valine at positions 10 and 11. ACE, a di-carboxy peptidyl peptidase with a molecular weight of 150 to 180 kDa, cleaves Ang I to generate Ang II by removing the last two amino acids of the decapeptide (see Table 5.1 ). Although the role of ACE in forming Ang II was first identified by Skeggs, Marsh, Khan, and Shumway in 1954, recognition of its critical role in the renin angiotensin system cascade awaited the development of the solid-phase method of peptide synthesis by Merrifield in 1964. Ng and Vance’s identification of the lung as a critical physiological locus for the processing of circulating Ang I into Ang II set the stage for the development of ACE inhibitors, the first landmark clinically successful approach to blockade of the renin angiotensin system.
ACE can act on other substrates, including bradykinin, substance P, and the tetrapeptide N-acetyl-Ser-Asp-Lys-Pro (Ac-SDKP). ACE-mediated inhibition of bradykinin and Ac-SDKP metabolism are implicated in contributing to the mode of action of ACE inhibitors through potentiation of bradykinin vasodilator responses or antifibrotic mechanisms. The occurrence of angioedema, a side effect of ACE inhibitors more frequent in women and black patients, has also been linked to prevention of bradykinin and substance P metabolism.
ACE is ubiquitously found in cardiovascular tissues, primarily expressed in epithelial and endothelial cells. ACE activity found in blood, lymph, and cerebrospinal fluid represents a form of the enzyme that has lost its anchoring to the cell’s membranes by the cleavage of its C-terminus hydrophobic region. The process accounting for the shedding of ACE from the vascular endothelium remains to be characterized. Recently, lysozyme and bilirubin have been identified as contributing to ACE-shedding. Another feature of ACE that bears importance for its role within the renin angiotensin system arises from the fact that its extracellular loop contains two catalytic sites termed the C- and N-domains. While the catalytic activity of the C-domain has been linked to the processing of Ang I into Ang II, the N-domain cleaves Ang-(1-7) into Ang-(1-5).
The variety of substrates on which ACE can express its hydrolytic activity implicate its role in the regulation of multiple organ and cellular functions. Since human kidneys contain large proportions of ACE compared with other organs other than the lung, it has been argued that renal ACE-mediated Ang II formation acts as a master switch in the control of nephron sodium transport and the development of hypertension.
Chymase
Chymases are a group of chymotrypsin-like serine proteinases that have multiple functions in tissue injury and remodeling. Phylogenetic studies have divided chymases into two isoenzyme groups, α and β. An α-chymase gene has been identified in all mammals, including humans, while β-chymases exist only in several rodent species. β-Chymases are not expressed in humans. This is an important distinction because α-chymase forms Ang II, while β-chymases can both form and degrade Ang II. Chymase is elaborated by mast cells, vascular endothelial cells, and other interstitial cells within the human heart. The identification of other cellular sources, including cardiac fibroblasts and vascular endothelial cells, demonstrates a more widely dispersed production and distribution system that accounts for its relatively high protein abundance (about 1.3 μg/g in human heart tissue). Evidence for its intracellular presence in cardiomyocytes in the human heart opens an entirely new compartment of chymase-mediated actions that were previously thought to be limited to the extracellular space.
Chymase is 20 times more catalytically efficient than ACE in Ang I conversion to Ang II in the human heart. With ACE largely located on endothelial cells with its catalytic site exposed to the vascular lumen and chymase activity largely located in tissue, there is a compartmentalization of ACE- and chymase-mediated Ang II formation in the tissue and vascular space. The presence of chymase in tissue has been invoked in the mechanism of “ACE escape” and in a more indirect means through ACE inhibitor increase in bradykinin, causing mast cell degranulation and chymase release. Most recently, AGT has been shown to be a substrate for Ang-(1-12) generation in the human heart that is independent of renin and ACE.
In addition to its very potent Ang II-forming capacity, chymase protease actions also mediate cleavage of matricellular proteins and peptides, including laminin and fibronectin, important in cell survival; chymase activates peptide and enzyme precursors including matrix metalloproteinases (MMPs), transforming growth factor-β (TGF-β), stem cell factor (SCF) (causing further mast cell degranulation and chemotaxis), interleukin-6 (IL-6) and IL-1β, and conversion of pre-pro-endothelin I (pre-proET1). All these mechanisms of action are crucial factors in cardiovascular injury and remodeling (see also Chapter 1, Chapter 2, Chapter 4, Chapter 12 ). Taken together, chymase mediates a critical balance of (1) profibrotic ET-1 and Ang II formation, myofibroblast formation, and TGF-β activation; (2) antifibrotic protease activation of MMPs, direct digestion of matricellular proteins, and formation of bradykinin (BK); and (3) inflammatory cytokines and SCF activation and chemotaxis of inflammatory cells. These multiple protease actions in addition to its Ang II-forming capacity are complemented by chymase upregulation in the human myocardial infarction heart and vulnerable atherosclerotic plaque, aortic aneurysm, atherosclerosis, and diabetic kidney.
Angiotensin-Converting Enzyme 2
ACE2 is a type 1 integral membrane glycoprotein that functions as a mono carboxypeptidase–converting Ang I into Ang-(1-9) and Ang II into Ang-(1-7). The affinity of the enzyme for Ang-(1-7) formation from Ang II is higher than its affinity for Ang I. Although ACE2 is a close ACE homologue, ACE2 catalytic activity is not blocked by drugs that inhibit ACE. Like many other enzymes, ACE2 displays hydrolytic activity for other proteins such as vasoactive bradykinin (1–8), des-Arg-kallidin, Apelin-13, and Apelin-36. Additional features of the enzyme include 48% sequence identity with collectrin at the noncatalytic C-terminal domain of the enzyme and the demonstration that ACE2 is the receptor for SARS virus. While ACE2 is most abundant in heart, kidney, the retina, and the utero-placenta complex, a secreted form of ACE2 generated from the hydrolytic activity of a disintegrin and metalloprotease domain 17 (ADAM17) is present in blood.
The Crackower et al. landmark study demonstrated ACE2’s role in the regulation of cardiac function. That Ang II influenced ACE2 gene expression in the heart provided evidence for the existence of a regulatory control of Ang II expression by the opposing actions of ACE as an Ang II-forming enzyme versus the ACE2 Ang II-degrading mechanism. Additional studies directed to understand the signaling mechanisms by which Ang-(1-7) acts as a negative regulator of Ang II actions identified a primary role of ACE2 in the formation of the heptapeptide from Ang II in the heart. These studies showed that cardiac overexpression of ACE2 reversed cardiac hypertrophy in SHR, identified the mas receptor as the site at which Ang-(1-7) acts to inhibit myocyte hypertrophy, demonstrated a role of ACE2 in modulating the central neurogenic actions of Ang-(1-7), and revealed a critical role of the ACE2/Ang-(1-7)/mas-receptor axis in the regulation of fetal development and pregnancy. These data now constitute the foundation for a more precise mechanistic analysis of the biological and signaling processes by which the ACE2/Ang-(1-7)/mas receptor axis counteracts the actions of Ang II on blood pressure, cardiac and vascular remodeling, and metabolic and renal function.
Other Angiotensin-Forming Enzymes
Because proteolytic enzymes participate in multiple regulated cellular processes, alternate biotransformation pathways within the renin angiotensin system need not be restricted to classic canonical and non-canonical pathways. The molecular basis for the effector hormonal and tissue actions of angiotensins in maintaining the normalcy of the internal environment transcends their function in the control of fluid volumes and tissue perfusion. The tissular actions of Ang II as a growth factor, a stimulant of fibrosis, and its prothrombotic mechanisms need not be identical in the heart, brain, kidneys, and bone marrow. The demonstration of the existence of renin angiotensin system genes in the intracellular environment need not reflect a functional processing of biologically active angiotensins through enzymatic pathways previously characterized in the circulation or even the extracellular compartments. Ang II participation in innate and adaptive immune mechanisms, modulation of hematopoiesis, and progenitor cell expression and function may result from biochemical biotransformation of precursor peptides where neither renin nor ACE play a role. In the context of this discussion, emerging studies demonstrating novel roles for carboxypeptidase A and B (see Table 5.2 ), elastase, cathepsin G, proteinase 3, caspase-1, and chymase in angiotensin peptide metabolism or processing of inflammatory cytokines such as IL-1β deserve future attention.
Main Angiotensin Products
The demonstration that the renin angiotensin system contains both a pressor and depressor arm is now well accepted, and the source of potential therapeutic approaches based on augmenting the activity of the depressor arm. Since these concepts were advanced by Ferrario’s laboratory, others have taken to exploring the dynamics of these mechanisms mostly in laboratory animals. The pressor arm of the system is defined as being composed of the enzyme ACE, the Ang II product, and the Ang II type 1 (AT 1 ) receptor (ACE/Ang II/AT 1 -R axis). The opposing arm of the system is defined as including the enzyme ACE2, the Ang-(1-7) product, and the mas receptor [ACE2/Ang-(1-7)/mas axis]. Since the original description of the two opposing arms by Ferrario, Ang-(1-9) cardio renal protective actions should be included as a component of the opposing arm of the system, while a resurgence of the importance of chymase as the major Ang II-forming enzyme in humans needs similar inclusion.
Angiotensin-(1-12)
There has been a paucity of research regarding the potential existence of intermediate peptides derived from AGT with molecular sequences larger than Ang I (see Fig. 5.2 ). Earlier studies identified low and high molecular forms of circulating AGT in plasma. A high-molecular-weight AGT is present in human plasma at concentrations amounting to no more than 5% of the total circulating substrate. The high-molecular-weight form of the substrate is increased in normal pregnancy and pregnancy-induced hypertension. In 2006, Nagata et al. reported the presence of proangiotensin 2 (currently renamed as Ang-[1-12]) in the plasma and tissues of a Japanese strain of Wistar rats. The peptide was shown to generate Ang II, as the pressor response induced by its injection into the systemic circulation could be abrogated by pretreatment with captopril or candesartan. The discovery of this extended Ang I form was confirmed to function as an endogenous source for Ang II production in rodent hearts and kidneys, human left ventricular tissue, and left atrial tissue obtained from humans undergoing heart surgery. Overall, the accumulating evidence demonstrates that Ang-(1-12) may be an intracellular substrate accounting for Ang II actions through the hydrolytic activity of chymase. A second peptide composed of 25 amino acids has been identified in human urine. Like Ang-(1-12), big angiotensin-25 (Ang-[1-25]) generates Ang II through the catalytic action of chymase. The existence of intermediate forms of AGT that generate Ang II independent of renin uncovers non-canonical mechanisms for interstitial or intracellular Ang II production.
Angiotensin-(1-9)
The nonapeptide Ang-(1-9) is a relatively new addition to the active renin angiotensin system effector molecules, possessing actions like those associated with Ang-(1-7) in terms of systemic vasodilation, cardiac hypertrophy, and hyperplasia. Ang-(1-9) actions are mediated through binding of the peptide to AT 2 receptors. Although there is a tendency to consider Ang-(1-9) as a product of Ang I by ACE2, Ocaranza et al. report a greater affinity of carboxypeptidase A and cathepsin A in processing Ang I into Ang-(1-9). Ang-(1-9) metabolism into Ang-(1-7) occurs via the action of ACE, although prolyl endopeptidase, neprilysin, and thimet oligopeptidase have been reported to have comparable hydrolytic actions. It is of interest to note that the antihypertensive cardio-renal protective actions of ACE inhibition may be amplified through inhibition of Ang-(1-7) and Ang-(1-9) metabolism. Since neurolysin (E.C. 3.4.24.16), a cytosolic enzyme, has moderate binding affinity for Ang-(1-9), it has been suggested that this mechanism may downregulate binding of Ang II to AT 1 -R. Ang-(1-9) has been detected in human blood and heart tissue, and the nonapeptide shows significant ability to stimulate atrial natriuretic peptide. Further work should be pursued to determine the potential ability of Ang-(1-9) to counteract the neurohormonal mechanisms accompanying chronic heart failure. Comparative studies of the action of Ang-(1-7) versus Ang-(1-9) are needed.
Angiotensin I and Angiotensin II
There is no evidence of biological actions of Ang I other than to serve as the substrate for the generation of Ang II via ACE in rodents and chymase in humans or Ang-(1-9) production from ACE2. Ang II biological actions, primarily mediated through binding of the agonist to AT 1 receptors, represent the major effector pathway of the renin angiotensin system with pleotropic physiological and pathological actions that contribute to chronic diseases, inflammation, metabolic abnormalities, and cancer. Ang II catabolism is mediated by aminopeptidases (angiotensinase A) or through processing of the peptide into Ang-(1-7) by ACE2. The hydrolytic activity of aminopeptidase A through the cleavage of Asp 1 in the amino-terminal end of the molecule accounts for the generation of Ang III, which is the preferred agonist of renal AT 2 receptors. Aminopeptidase N converts Ang III into Ang IV. Thus aminopeptidase activities can have significant effects on the catabolism of the amino terminal end of Ang II.
Angiotensin III and Angiotensin IV
Since the pioneer studies of Goodfriend and Peach, the heptapeptide [des-Asp 1 ] Ang II (angiotensin III; see Table 5.2 ) has been reported to reproduce all of Ang II effects, although with lower potency. Studies exploring Ang III mechanisms of action in the brain suggested that Ang II needed to be converted into Ang III for selective binding to AT 1 receptors (Ang III hypothesis) . No conclusive evidence in support of this hypothesis has become available. On the other hand, recent studies demonstrate that Ang III inhibits renal proximal tubule sodium (Na + ) reabsorption by AT 2 receptors. These studies affirm a natriuretic role for renal AT 2 receptors. Whether similar differential actions of Ang III versus Ang II in other organs exist remains undetermined, although Ang III was shown to induce phosphorylation of extracellular signal-regulated kinases (ERK1/2) and stress-activated protein kinase (SAPK)/c-jun N-terminal kinase (JNK) pathways with a potency comparable to Ang II.
Ang IV is another peptide fragment, with a truncated amino terminal end that was originally thought to bind to another G-couple subtype angiotensin receptor (AT 4 ). In-depth studies of its actions in neural tissues revealed the specific, high-affinity binding site to be the insulin-regulated aminopeptidase (IRAP), a protein highly expressed in vesicles containing the insulin-sensitive glucose transporter GLUT4. A soluble form of IRAP is reported to degrade oxytocin and vasopressin. Main biological actions of Ang IV appear to target the brain, where the hexapeptide enhances learning and memory. While Ang IV may act as a weak vasodilator agonist in the circulation, it is able to augment renal blood flow and inhibit tubular sodium transport.
Angiotensin-(1-7)
The 1988 demonstration that a heptapeptide fragment of Ang I, lacking the sequence of Phe 8 -His 9 -Leu 10 (see Table 5.1 ), was biologically active accounts for the radical change of thinking regarding the biochemical physiology and function of the renin angiotensin system. Characterization of the biochemical pathways responsible for the formation of Ang-(1-7) from Ang I revealed the role of several tissue-specific endopeptidases prolyl oligopeptidase 24.26 (brain, vascular endothelium, and kidney), neutral endopeptidase 24.11 ([neprilysin] circulation and kidney), and metalol-endopeptidase 24.15 (brain, vascular smooth muscle). The activity of these enzymes in Ang-(1-7) formation is dependent in part on the relative concentration of the enzyme in tissues, since in the kidney neprilysin plays a predominant role in the hydrolysis of Ang-(1-7) into Ang-(1-4). In contrast, ACE cleaves Ang-(1-7) into Ang-(1-5) in the circulation, the vascular endothelium, and the lung. There is now agreement that Ang-(1-7) binds to the mas receptor in conveying the signal to account for most of Ang-(1-7)’s biological actions.
While Ang-(1-7) was initially shown to be a vasodilator peptide functioning to oppose Ang II vasoconstrictor actions (see Fig. 5.1 ), Ferrario and colleagues first advanced the hypothesis that hypertension and aging, as well as impaired cardiac, renal, vascular, and metabolic function, was the result of a deficiency in the expression of the ACE2/Ang-(1-7)/mas-receptor axis rather than simply an increased expression of Ang II pressor and proliferative actions. This hypothesis was buttressed by original studies showing low urinary excretion rates of Ang-(1-7) in untreated essential hypertensive subjects restored by chronic treatment with an ACE inhibitor or a dual vasopeptidase inhibitor. A turning point in the validation of Ang-(1-7) critical counterregulatory function was achieved by the physiological and molecular characterization of the role of ACE2 in regulating Ang-(1-7) endogenous production from Ang II. Ang-(1-7)’s mechanism of action in terms of inhibiting myocyte hypertrophy, exhibiting antiproliferative actions, counteracting inflammatory signals and expression of ROS, facilitating nitric oxide (NO) release, and inhibiting platelet aggregation and low-density lipoprotein cholesterol deposition in the subendothelial region of large arterial vessels are summarized in multiple publications. Signal transduction pathways in which Ang-(1-7) acts as a ligand are complex, engaging not only the mas receptor but also through the binding of the heptapeptide to AT 2 -R and possibly bradykinin B2 receptors. Since the original studies by Loot et al., Ang-(1-7) has been reported to act as a cardioprotective agent in myocardial infarction and heart failure. Newer studies document that Ang-(1-7) restores myocyte L-type calcium current (ICa ++ ,L) responses in experimentally induced heart failure through a mas receptor signaling mechanism involving activation of NO/bradykinin pathways. Ang-(1-7) increases in (Ca ++ )I transients induces positive inotropic and lusitropic effects in left ventricular function and myocyte contractility in heart failure.
Ala 1 -Angiotensin II and Almandine
An intriguing 2007 investigation reported the detection of an Ang II-like peptide in human plasma, in which the amino acid Aspartic acid (Asp) had been replaced by Alanine (Ala) in position 1 of the N-terminus of the molecule. The peptide, named angiotensin A (Des[Asp 1 ]-[Ala 1 ]-Ang II; Ang A), possessed biological activity comparable to Ang II but with a higher affinity for AT 2 receptors. On the other hand, the active metabolite of losartan (EXP-3174) abolished the vasoconstrictor response induced by Ang A, while PD123319 (AT 2 receptor antagonist) had no effect. The discrepancy between high Ang A AT 2 receptor affinity, and persistence of a biological effect following blockade of this receptor remains unexplained. Ang A formation was interpreted by Jankowski et al. to be the result of the enzymatic activity of mononuclear leukocyte-derived aspartate decarboxylase.
In the steps of the German investigators, a group of researchers working under the tutelage of the Brazilian investigator Robson Santos reported in 2017 a comparable decarboxylase process that results in the formation of a (Des[Asp 1 ]-[Ala 1 ]-Ang-[1-7]). The heptapeptide Ala 1 -Ang-(1-7) is named almandine. Fig. 5.2 outlines the various proposed pathways associated with the generation of almandine. Another potentially distinctive feature of almandine is that the transformed heptapeptide appears to show higher affinity for a subclass of mas-related proteins, the MrgD receptors. The mas-related G-protein coupled receptor member D (see Table 5.2 ) is a protooncogene previously associated with functioning as a specific receptor for beta-alanine. The significance of Ang A and almandine as secondary tissue-alternates regulating the cardiovascular actions of the renin angiotensin remains to be understood.
Angiotensin II Mediated Signaling Pathways
Angiotensin II Receptors
Ang II exerts its biological function through two major Ang II receptors: AT 1 -R and AT 2 -R. AT 3 -R and AT 4 -R are also described in cardiovascular organs. Since their biological functions are not well characterized, this chapter will focus primarily on the signaling pathways transduced by AT 1 -R and AT 2 -R. While AT 1 -R is widely expressed in many organs including kidney, brain, heart, and vasculature, expression of AT 2 -R in healthy subjects declines in these tissues after birth but could be upregulated under pathological conditions. Both receptors are seven transmembrane receptors and belong to the superfamily of G protein-coupled receptors (GPCR), sharing only 34% sequence identity. In general, AT 1 -R mediates Ang II regulation of blood pressure, cell growth and proliferation, cardiac contractility, and salt/water balance. However, an excessive Ang II stimulation of AT 1 -R results in a variety of deleterious cardiovascular effects reflected by AT 1 -R-mediated vasoconstriction, hypertrophy, apoptosis, fibrosis, oxidative stress, inflammation, enhancement of sympathetic outflow, and aldosterone release. On the other hand, a growing body of evidence testifies that activation of AT 2 -R counterbalances the effects of Ang II on AT 1 -R inducing vasodilation, decreasing cell proliferation, and fibrosis. It is also suggested that Ang II action on unopposed AT 2 -R may contribute to the beneficial cardiovascular effects of AT 1 -R pharmacological blockade. Additionally, inhibition of AT 1 -R signaling may be also caused by dimerization of the two receptors. However, the significance of AT 2 -R-mediated effects in cardiovascular pathology still remains unclear, since conflicting studies point to their deleterious cardiovascular effects as well.
Besides AT 1 -R/AT 2 -R interactions, these receptors may undergo dimerization with other receptors as well. It has been suggested that heterodimerization with the bradykinin B 2 or adrenergic receptors potentiates AT 1 -R signaling. Importantly, valsartan, an AT 1 -R antagonist, blocks signaling pathways of both AT 1 -R and adrenergic receptors in mice, whereas β-adrenergic receptor blockers restrict Ang II signaling cascade in heart failure. AT 1 -R can also heterodimer with apelin, mas, and several dopamine receptors to alter receptor signaling. On the other hand, AT 2 -R may dimer with the mas receptor, and AT 2 receptor blockers may cancel beneficial effects of mas activation in the brain of hypertensive animals. Moreover, to enhance their biological activities, both Ang II receptors can form homodimers before being translocated to the cell membrane.
Like many GPCRs, Ang II mediated AT 1 -R activation is subject to desensitization and internalization due to decreased receptor responsiveness. In this process, several serine/threonine residues on the cytoplasmic part of the receptors undergo phosphorylation by G-protein receptor kinases (GRK). Ang II can also phosphorylate AT 1 -R, providing a mechanism of how chronic Ang II activation of AT 1 -R downregulates its own receptor. Following phosphorylation, recruitment of β-arrestin dissociates AT 1 -R from G proteins and starts receptor internalization into clathrin-coated pits or noncoated specialized microdomains called caveolae. Most recently, it has been demonstrated that β-arrestin has a role not only in heterologous AT 1 -R desensitization, but also in mediating G protein-independent signaling of AT 1 -R (see also Chapter 13 ). These findings reveal an additional layer of GPCRs interaction without forming receptor heteromers. Most of the internalized receptors are degraded in lysosomes, while roughly one-fourth of them recycle to the plasma membrane. A recent study showed that AT 2 -R do not undergo desensitization due to the lack of ability to recruit β-arrestin. Thus it is possible that, upon activation, AT 2 -R may mediate a prolonged signaling response.
Ang II interaction with AT 1 -R involves G protein-dependent and G protein-independent signaling cascades that trigger a series of pathophysiological effects of Ang II. AT 1 -R mostly couples with G proteins (Gαq/11, Gαi/o, Gα12/13) to induce classic G-protein mediated pathways. The AT 1 -R-coupled G-protein mediated signaling also involves transactivation of growth factor receptors, as well as activation of several tyrosine kinases, mitogen-activated protein kinases (MAPKs), small GTP-binding proteins, and nicotinamide adenine dinucleotide phosphate (NADPH) oxidases (NOXs). In addition, AT 1 -R signaling involves G protein-independent pathways as well, through activation of tyrosine kinases and β-arrestin recruitment. It is important to note that the later mechanism may represent a cardioprotective signaling response. On the other hand, the beneficial cardiovascular effects of AT 2 -R predominately reflect its coupling to Giα, leading to activation of protein phosphatases and the NO-cyclic guanosine monophosphate (cGMP) system.
Ang II/AT 1 -R-Mediated G Protein-Coupled Pathways
Ang II activation of AT 1 -R involves dissociation of heterotrimeric G proteins (Gα, Gβγ) by replacing guanosine diphosphate (GDP) in the Gα subunit with guanosine triphosphate (GTP). There are several subfamilies of Gα and Ang II/AT 1 -R signals through Gαq/11, Gαi/0, and Gα12/13. The Gβγ complex also transduces Ang II/AT 1 -R cellular effects. In addition to GTPase, which restores the original association of the inactivate states of Gα and Gβγ complex, several GRKs and regulators of G protein signaling (RGS) contribute to the restriction of GPCR activation and may be potential targets for novel therapeutic approaches.
Ang II/AT 1 -R-Mediated Classic G Protein-Coupled Signaling Pathways
Ang II/AT 1 -R interaction involving Gαq/11 protein activates several signaling cascades important for regulation of blood pressure, cardiac growth, and contractility ( Fig. 5.3 ). One of the Gαq/11-mediated downstream signaling pathways involves activation of phospholipase C (PLC-β), leading to hydrolysis of phosphatidylinositol-4,5-bisphosphate (PIP 2 ) into two key second messengers: inositol trisphosphate (IP3) and diacylglycerol (DAG). IP3, binding to its receptor on the sarcoplasmic reticulum, releases Ca 2+ into the cytoplasm. Additional Ca 2+ influx resulting from Ang II coupling to Gα12/13 and activation of plasma membrane L-type Ca 2+ channels further promotes Ca 2+ binding to calcium binding proteins, calmodulin/troponin, and activation of myosin light chain kinase (MLCK). Phosphorylation of the myosin light chain by MLCK enhances the interaction between actin and myosin, promoting vascular and cardiac contraction. Negative regulation of contractility is imposed by myosin light chain phosphatase (MLCP), which deactivates MLCK. In contrast, MLCP inhibition by Rho kinase results in enhanced contraction.
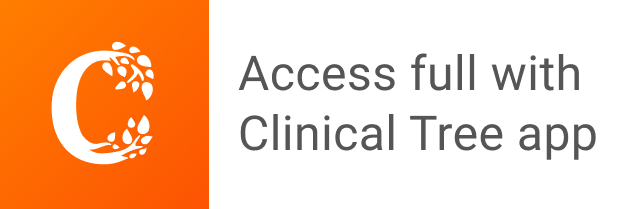