INTRODUCTION
Heart failure is an ancient diagnosis. Some of the earliest known descriptions are attributed to Hippocrates, circa 460–370 BCE. He reported dozens of clinical case histories with examples of dyspnea, as seen in left heart failure, and dropsy, as seen in right heart failure. Over the centuries, it has become apparent that the symptoms of heart failure are the result of complex pathophysiologic processes that alter both anatomic and physiologic properties of the heart.
In the United States alone, approximately 5 million people are afflicted by heart failure, with an annual incidence of 500,000 new diagnoses and 300,000 deaths. One third of patients with symptomatic heart failure have a normal ejection fraction, and only in the last decade has there been a shift in the paradigm, focusing attention on diagnosing and understanding diastolic dysfunction. In many cases, diastolic dysfunction is caused by one or more abnormalities of cardiac structure, such as hypertrophy, fibrosis, infiltrative disease, or pericardial constriction. However, many patients appear to have diastolic dysfunction due to cellular abnormalities of myocyte relaxation, which is reversible and transient and occurs mainly in the setting of ischemia or hypoxia. Other causes include cellular calcium overload or ATP depletion. Metabolic processes such as alkalosis, cardiovascular drugs, and the hypertrophy process itself can also alter the contractile and metabolic phenotype.
This chapter will focus on the cellular mechanisms of normal and impaired myocardial relaxation. Calcium plays a crucial role in this process. Its movement into the cytosol, caused by excitation at the surface membrane, stimulates contraction. This physiologic phenomenon is appropriately termed excitation-contraction coupling. The reverse of this process, which is dependent on the movement of calcium and sodium, can be thought of as repolarization-relaxation coupling. In normal hearts, sympathetic stimulation can increase contractility by exerting a positive inotropic effect as part of the excitation-contraction (E-C) process and can also increase the rate of relaxation, a positive lusitropic (relaxation-enhancing) effect, by facilitating Ca 2+ removal from the sarcoplasm. Myocyte control of relaxation occurs through the regulation of calcium concentrations within the cytosol via four main pathways involving sarcoplasmic reticulum (SR) Ca 2+ -ATPase, sarcolemmal Na + /Ca 2+ exchange, sarcolemmal Ca 2+ -ATPase, or mitochondrial Ca 2+ uniport. The latter two pathways have been studied and appear to have no significant effect on the beat-to-beat regulation of intracellular calcium.
We will discuss the other two mechanisms in detail along with myofibrillar calcium responsiveness, as much research is being directed in trying to develop novel therapeutic interventions targeted toward repolarization-relaxation coupling in an effort to maximize positive lusitropic effects.
PATHOPHYSIOLOGY
Excitation-Contraction and Repolarization-Relaxation Coupling
The E-C coupling process activates contraction by coupling the signal generated by the action potential (excitation) at the myocyte cell surface to the delivery of calcium into the cytosol that initiates contraction. After spontaneous depolarization of the surface membrane, Ca 2+ enters the myocyte through voltage-gated L-type channels (which are dihydropyridine receptive and will be referred to as DHRs). This influx serves as a trigger for the release of stored Ca 2+ from the SR via Ca 2+ release channels called ryanodine receptor 2 (RyR2). This process is known as calcium-induced calcium release (CICR). The transverse tubule system, unique to the mammalian heart, allows for the close proximity of the DHRs to clusters of RyR2. The initial influx of calcium through L-type channels activates the RyR2 channels within its domain in a synchronized fashion, spontaneously increasing local concentration of calcium and producing a Ca 2+ spark (named according to its appearance by confocal microscopy), which can also be thought of as a local Ca 2+ transient. During E-C coupling, several thousand Ca 2+ sparks occur in synchrony, overlapping in time and space, thus allowing for a global and uniform calcium transient that is sufficient for myocardial contraction. Another channel within the SR membrane, inositol triphosphate, has been reported to induce the release of calcium, but the rate and extent of release is much lower, and it is not triggered by CICR.
It is important to understand the cardiac contractile apparatus from the vantage point of troponin-C, the Ca 2+ receptor protein. When cytosolic calcium levels are low, there is minimal or no calcium bound to troponin-C. The troponin-tropomyosin complex inhibits the formation of the actomyosin complex. Once calcium is released from the SR, Ca 2+ binds to troponin-C, changing the configuration of the troponin-tropomyosin complex, removing the inhibition of the actin-myosin interaction, and thus allowing cross-bridge cycling and contraction.
Another important messenger that modulates E-C coupling is cyclic adenosine monophosphate (cAMP). This pathway is initiated by activation of the β-adrenergic receptors, which then activate adenylate cyclase and generate cAMP. This nucleotide activates a series of phosphorylating enzymes (i.e., protein kinases). Protein kinase A (PKA) binds to A-kinase anchoring proteins (AKAPs) and phosphorylate calcium regulatory proteins at multiple subcellular sites. Phosphorylation of both the voltage-gated L-type channels and RyR2 channels (Ca 2+ release channels) leads to a net effect of increased Ca 2+ entry and a greater calcium release (a function of the amount of Ca 2+ stored) from the SR, producing an increase in the rate and magnitude of force generation (positive inotropic effect). This is balanced by the phosphorylation of phospholamban on the SR, which regulates the sarcoendoplasmic reticulum Ca 2+ (SERCA)-ATPase pump and allows for greater reuptake of calcium from the cytosol, enhancing the rate of relaxation (positive lusitropic effect). Finally, the phosphorylation of troponin-I, part of the regulatory complex of the contractile apparatus, facilitates the dissociation of calcium from troponin-C by altering the myofilament calcium sensitivity, also causing a positive lusitropic effect. These changes increase the amplitude of the systolic Ca 2+ transient and decrease its duration, thus increasing myocyte contractility and generation of force. When the β-adrenergic pathway is activated in times of increased cardiac output demand, there is an increased heart rate and simultaneous increase in force (positive force frequency) and an increase in rate of relaxation, which ensures that sufficient calcium is available from the SR for the next beat.
The Failing Human Heart
In the failing human heart, adrenergic effects are blunted. Numerous studies of mammalian heart models have been done to better understand the mechanism that leads to abnormal adrenergic signaling. The normal human atrial and ventricular myocardium expresses β1- and β2-adrenergic receptors at a ratio of about 70:30. In the early stages of heart failure, there is an increase in sympathetic stimulation in order to maintain adequate blood pressure and cardiac output. As the syndrome progresses, the continuous activation eventually leads to a downregulation of β1 receptors by almost 60% to 70% and desensitization of the remaining receptors, which depresses cAMP levels, hence contributing to altered calcium regulation and reducing intracellular calcium levels. The overall effect is a depressed and negative force-frequency relationship. In vitro studies of nonfailing and failing human myocytes have been done of contractility under varying conditions (i.e., varying muscle length and loading conditions as seen with pressure or volume changes in vivo, slow rates, [Ca 2+ ], and catecholamine stimulation). These studies concluded that during basal conditions, the contractile properties of normal and failing myocytes is similar. Conversely, the same is not true during increased inotropic stimulation. The normal myocyte is able to increase force generation, but in failing myocytes, the developed force either decreases or remains unchanged. Therefore, during low workload states, contractility is preserved, but “contractility reserve” (the ability to increase contractility with heart rate or sympathetic stimulation) is severely reduced in failing myocytes. The proposed mechanism at this time is that the amount of calcium that is released by the SR of failing myocytes is less than in normal myocytes, which may be due to decreased SR Ca 2+ stores or abnormal SR Ca 2+ loading ; however, there is a need for more investigational studies to fully understand the mechanisms behind the E-C coupling defects that contribute to dysfunctional Ca 2+ handling.
Abnormal Calcium Regulation in Heart Failure
Abnormal modulation of intracellular calcium is a major mechanism that underlies both systolic and diastolic dysfunction and develops with cardiac hypertrophy and failure. The heart spends more than half of its time in diastole (i.e., relaxation and filling), yet there is controversy in the definition of diastolic dysfunction and diastolic heart failure. Abnormal calcium regulation comprises a spectrum of changes that include slowed force (or pressure) decline and cellular re-lengthening (increased ventricular stiffness), increased (or decreased) early filling rates and deceleration, an elevated diastolic pressure-volume relationship, and a filling-rate–dependent pressure elevation (increased end-diastolic pressure). These changes occur due to abnormal regulation of Ca 2+ homeostasis within the myocyte. Although the cellular and molecular regulation of calcium in failing myocardium has been studied, the exact mechanism of abnormal calcium regulation is still not well understood ( Fig. 1-1 ).

Normally, the systolic calcium transient occurs with the release of calcium from the SR, which is triggered by the L-type channel Ca 2+ influx. The magnitude of calcium release is dependent on the Ca 2+ influx and the amount of calcium stored in the SR. Calcium reuptake is mediated by the SR Ca 2+ -ATPase pump and the sarcolemmal Na + -Ca 2+ exchanger (NCX), which results in the decay of the Ca 2+ transient and normal relaxation. In failing myocytes, there are numerous changes (e.g., expression levels of these membrane proteins), which result in abnormal Ca 2+ transient, particularly in its termination. This also causes a prolonged action-potential duration and delayed relaxation. At slow pacing rates, the Ca 2+ transients of normal and failing myocytes are very similar. However, when the heart rate increases, the Ca 2+ transient becomes significantly different due to a negative force-frequency relationship and decreased SR Ca 2+ release, as discussed earlier.
Sarcolemmal Receptors and Mechanisms
The cardiac sarcolemma is a complex structure that contains multiple channels, exchangers, and pumps that are necessary for normal E-C coupling and myocyte contraction. In recent years, the sarcolemmal NCX has surfaced as one of the primary agents necessary to extrude calcium with each heart beat to allow normal relaxation. By virtue of the NCX mechanism, the role of the electrochemical sodium gradient has also been studied in various mammalian species as a potential determinant of [Ca 2+ ] i .
The NCX is a bidirectional electrogenic ion transporter that utilizes the Na + electrochemical gradient to exchange one calcium for three sodium ions. During repolarization, the negative membrane potential and elevated [Ca 2+ ] i drive the NCX toward a forward mode (Na + in/Ca 2+ out), resulting in extrusion of calcium from the cell in diastole. When the membrane potential is positive ([Na + ] i is increased), the NCX functions in reverse mode (Na + out/Ca 2+ in), resulting in calcium influx, which may help regulate SR Ca 2+ load and also, in conjunction with the Ca 2+ current induced by activated DHRs, regulate SR Ca 2+ release. There still remain some conflicting data in regard to the effects of CICR on SR by the reverse mode I NaCa . CICR occurs mainly in the dyadic cleft space in the T-tubular regions.
Several studies using the detubulation method in rat ventricular myocytes concluded that a majority of the NCX was localized to the T-tubules. However, Scriven et al. studied the distribution of these proteins using high-resolution imaging and showed that the NCX was localized to areas outside of the dyadic cleft space and that the spatial proximity of DHR with RyR2 was higher than with NCX ; therefore, any triggered SR Ca 2+ release by the reverse mode I NaCa seems highly inefficient.
Several animal models with myocyte hypertrophy demonstrated elevated [Na + ] i compared with normal. Pieske and Houser measured [Na + ] i in failing and nonfailing human myocytes using multiple techniques and were the first to report elevated [Na + ] i levels in failing human myocytes. The exact mechanism of this is under further investigation. Several studies have tried to describe the processes that lead to Na + influx in the failing myocyte and the role of this influx in governing calcium homeostasis. One proposed mechanism suggests that sodium influx occurs through sodium channels and raises levels within the Na + microdomain activating the NCX in reverse mode (Ca 2+ influx), which is complementary to the influx generated by L-type calcium channels. Because the NCX is dependent on the Na + electro-chemical gradient for functioning, any changes that occur in sodium regulation in heart failure, when taken to an extreme, can result in [Ca 2+ ] i overload and diastolic dysfunction. Few studies have addressed the role of the Na + -H + exchanger in failing myocytes, which has a 1:1 stoichiometry of Na + influx for H + efflux. Its stimulation may be increased in heart failure and lead to elevated [Na + ] i . Decrease in intracellular pH (which increases H + ) can also increase Na + by means of the Na + -H + exchanger, secondarily increasing Ca 2+ by means of the NCX. Alteration of these exchanges may contribute to prolongation of the action potential, slowed decay of the Ca 2+ transient, and a delayed relaxation in failing myocardium.
Several models of heart failure and hypertrophy have also shown an increase in expression of NCX, which may be partially controlled by the decreased sympathetic stimulation, leading to decreased SERCA activity. Terraciano et al. showed that in myocytes from transgenic (heterozygous) mice with upregulation of NCX, there was an increase in reverse-mode function resulting in an increase in SR calcium stores compared with wild-type myocytes. It is important to remember that in smaller mammals (mice and rats), the action potential is shorter than the duration of the calcium transient, which means that repolarization is occurring during most of the calcium transient, favoring forward-mode NCX. In these species there are lower levels of NCX, so any efflux via NCX makes a very small contribution to the decay of the calcium transient, even when overexpressed. However, there is a high [Na + ] i in smaller mammals, favoring Ca 2+ entry by reverse mode during the latter part of the calcium transient, which becomes more pronounced in myocytes overexpressing NCX, explaining the findings reported by Terraciano’s group.
In normal human myocytes, the action-potential duration is prolonged, and SERCA release and reuptake occur during the plateau phase, when NCX is not in forward mode, indicating that most of the elimination of cytosolic calcium is dependent on reuptake by the SERCA pump. However, in failing myocardium, the interaction between these two proteins changes due to an increase in the ratio of NCX to SERCA levels. To better understand whether increased NCX activity in the setting of reduced SERCA activity affects diastolic function, a study discriminating failing human hearts into three groups based on diastolic dysfunction with increased stimulation rate was performed. The investigators discovered three different phenotypes with varying expression of SERCA and NCX with impaired systolic function, but the overall trend in the ratio of NCX to SERCA was an increase by a factor of 2 to 4 in all groups of failing myocytes compared with normal. The phenotypes at either end of the spectrum ranged from increased levels of NCX and unchanged SERCA levels (group I) to decreased levels of SERCA and unchanged NCX levels (group III). Only the latter phenotype demonstrated both systolic and diastolic dysfunction, suggesting that both SR calcium uptake and the capacity to eliminate calcium from the cytosol are impaired, whereas in group I, the SR calcium uptake is impaired (causing systolic dysfunction), and global capacity to eliminate calcium is higher (preserving diastolic dysfunction). Therefore, the overexpression of NCX has positive correlation with diastolic function in failing myocytes.
Sarcoplasmic Reticulum
The SR is an intracellular structure that is the most important store of calcium in the mammalian heart. The sarcoplasmic membrane proteins (RyR2 and SERCA) maintain a tight control of calcium release and uptake in E-C coupling, contractility, and relaxation. There is a 10,000-fold Ca 2+ gradient maintained across the SR membrane by the SR Ca 2+ -ATPase pump. Molecular analysis has identified three homologous genes (SERCA1, SERCA2, and SERCA3) encoding the SERCA pumps. The SERCA2 gene is spliced into four variants that encode the isoforms. SERCA2a is the primary isoform expressed in cardiac muscle at high levels; however, there are regional differences, age-related effects, and variation due to thyroid hormone levels that affect expression levels. Experimental models in animals and humans have demonstrated that the expression level of SERCA in the atrium versus the ventricle is twofold and may account for shorter contraction time in atria versus ventricles. In fact, in heart failure models, it is well established that defective SR Ca 2+ uptake correlates with decreased contractility, which could be attributed to significant decline in SERCA protein levels or an alteration in SR Ca 2+ transport function.
Several studies induced left ventricular pressure overload hypertrophy/failure in rats by thoracic aortic banding and consistently found an overall decrease in SERCA mRNA levels, suggesting that downregulation of SERCA2a gene expression in these models partly occurs at the transcriptional level. Feldman et al. also deduced that decreased SERCA mRNA levels could be a marker of transition from compensated hypertrophy to decompensated hypertrophy/failure. In human heart models, SERCA mRNA levels are reduced in failing compared with nonfailing hearts, yet there remains controversy regarding simultaneous decrease in SERCA protein expression. Recently, data from larger studies have successfully shown a reduction of SERCA protein levels in failing human hearts, but not in compensated hypertrophied human hearts, suggesting that perhaps a decrease in protein level is a sign of developing failure. A decrease in SERCA2a expression at the level of mRNA or protein is inversely related to duration of cardiac contraction, correlates with decreased myocardial function, alters the force-frequency response, and may also slow the velocity of relaxation, suggesting the importance of SERCA pump level in maintaining myocardial function. Yet it is still difficult to describe the actual relationship between cardiac muscle and the SERCA pump because of the complexity of its regulation and all the changes in other calcium-regulating proteins (such as NCX) that occur in concert with each other within the myocyte and the heart as the syndrome of heart failure progresses.
The SERCA pump is modulated by both direct and indirect factors. Phospholamban is the primary indirect regulator that activates the SERCA2a pump. In its dephosphorylated state, phospholamban inhibits SERCA2a affinity for calcium. The phosphorylation of phospholamban can occur at three different sites—serine-16 by cAMP-dependent PKA, threonine-17 by Ca 2+ /calmodulin-dependent protein kinase II, and serine-10 by Ca 2+ -activated phospholipid-dependent protein kinase. When phospholamban is phosphorylated by cAMP-dependent PKA (the most important mediator), the inhibitory effect is removed and the calcium affinity (not the maximal velocity of SERCA2a) increases, resulting in enhanced relaxation and an increase in SR Ca 2+ load. Ca 2+ /calmodulin-dependent protein kinase II (CaMK II) is the other modulator, which directly phosphorylates SERCA2 and increases V max (maximal activity) without altering the calcium affinity of SERCA2. CaMK II also phosphorylates the threonine-17 site in phospholamban, which also increases the calcium affinity of SERCA2a. In heart failure, the blunting of the β-adrenergic pathway leads to alteration not only in phosphorylation of phospholamban at the serine-16 site, but also in CaMK-dependent phosphorylation, ultimately altering SERCA2a activity. Most studies of human heart failure have suggested that although there is a decrease in phospholamban mRNA levels, there is no difference in protein expression between failing and nonfailing myocytes. Therefore, protein expression of SERCA2a in relation to phospholamban is always diminished in heart failure, which may explain the increased phospholamban-to-SERCA2a ratios, leading to an increase in inhibition of the SERCA2a pump and an overall decrease in its basal activity level and contributing to abnormal calcium handling.
Altered Calcium Responsiveness of Myofibrillar Tension
Cardiac contractility and relaxation are altered not only because of changes in calcium availability, but also because of changes in myofilament responsiveness to calcium. In fibers rendered hypermeable to Ca 2+ , a change in responsiveness can manifest as either a change in sensitivity or potency or as maximal Ca 2+ -activated force. The actual mechanism responsible for this effect has not been definitively determined, but based on numerous studies, it appears that isoform composition and phosphorylation status of the contractile proteins are altered, which increases the Ca 2+ sensitivity of the contractile apparatus in end-stage heart failure. Most studies have concentrated on changes of a single factor. Van der Velden et al. focused on a combination of contractile protein changes that occur during heart failure by studying isometric force and its Ca 2+ sensitivity in left ventricular myocytes from nonfailing and end-stage failing donor hearts. They concluded that the combined decrease in phosphorylation status of troponin-I and myosin light chain 2 resulted in an increase in Ca 2+ sensitivity, and not due to contractile protein isoform change. However, other studies have shown that the phosphorylation of myosin light chain 2 increases Ca 2+ responsiveness. Earlier reports showed that end-stage heart failure in humans is not associated with myofibrillar Ca 2+ sensitivity. Until now, no real consensus has been reached to explain if, why, and how Ca 2+ sensitivity increases in heart failure patients.
Therapeutically, Ca 2+ sensitizers pose a problem due to the mechanism of action. An increased affinity of Ca 2+ for troponin-C would enhance the actin-myosin interaction, which, theoretically, prolongs relaxation. This has been shown in vivo and in vitro in animals and in humans.
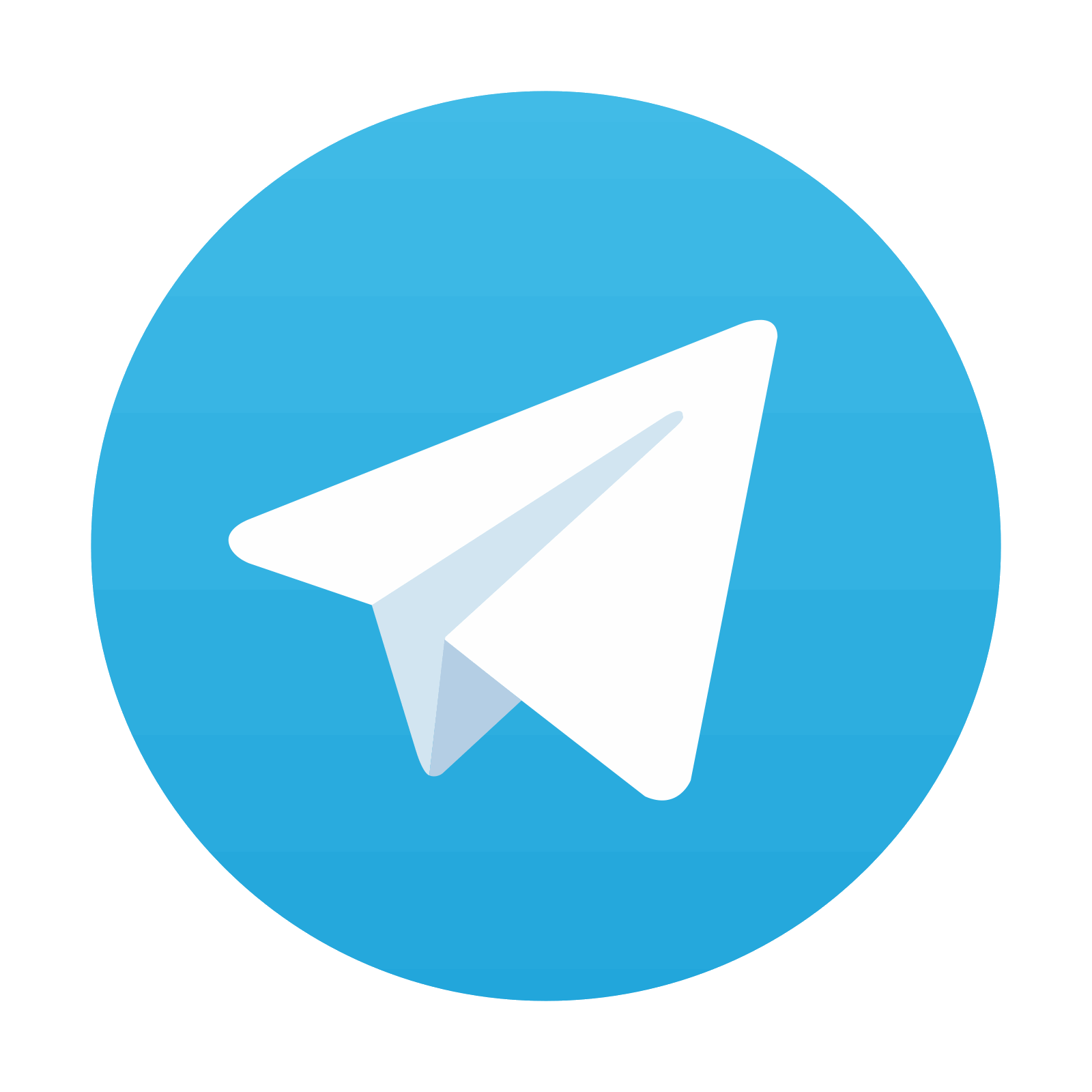
Stay updated, free articles. Join our Telegram channel
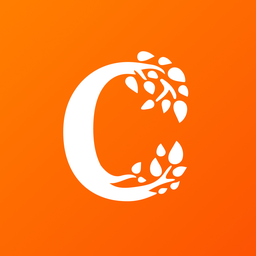
Full access? Get Clinical Tree
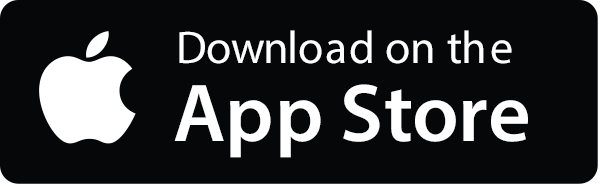
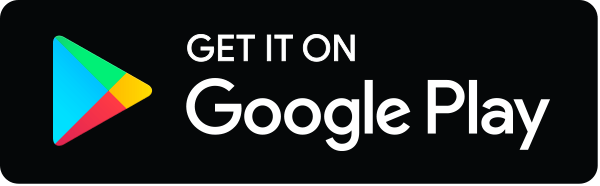