INTRODUCTION
Pulsed-wave Doppler velocities of mitral inflow are the most commonly used indices of diastolic function. Their application is nevertheless limited by their load dependency. Flow propagation (Vp) using Doppler color M-mode (CMM) has been proposed as a complementary technique to evaluate left ventricular (LV) relaxation.
Color Doppler M-mode echocardiography provides a spatiotemporal map of blood distribution (v(s,t)) within the heart, with a typical temporal resolution of 5 ms, a spatial resolution of 300 microns, and a velocity resolution of 3 cm/s. By aligning the cursor parallel to the streamlines of flow, we can observe the propagation of flow from the mitral valve to the apex ( Fig. 11-1 ). Assessment of diastolic flow propagation has offered novel information about LV filling dynamics and has been applied in a variety of clinical conditions. Since the initial descriptions by Jacobs and later Brun, computer simulation, in vitro modeling, and animal and clinical studies have improved our understanding of the determinant of Vp but also have shown the complexity of this index. Vp appears to be relatively independent of loading conditions and therefore may overcome one of the main limitations of Doppler-based techniques.

In this chapter we will review the current understanding of how flow propagates from the base to the apex of the left ventricle, its major determinants, how to properly obtain a color M-mode tracing, and clinical situations in which this technique may be applied. We will also describe its application in the calculation of intraventricular pressure gradients (IVPGs).
BACKGROUND
It is now well recognized that in early diastole small but significant intraventricular mitral-to-apex pressure gradients are generated. These IVPGs are related to elastic recoil and apical untwisting. They represent one of the driving forces of the blood entering the left ventricle (ventricular “suction”). As a result, when the mitral valve opens, a column of blood accelerates from the atrium toward the ventricular apex. In normal ventricles, flow propagates very rapidly, while in dilated, poorly contracted ventricles it occurs comparatively slowly ( Fig. 11-2 , right).

Mitral inflow propagation displayed by CMM has a complex pattern. The earliest CMM velocities often occur during isovolumic relaxation. After the mitral valve opens, there is a rapid initial component (phase I), often followed by a slower component (phase II). Finally, the last component in late diastole is associated with atrial contraction (see Fig. 11-1 ). Computer and in vitro modeling have studied the phenomenon of flow propagation. During phase I, blood moves almost simultaneously in the whole left ventricle, behaving as an incompressible fluid column (columnar flow). Phase II is a flow wave thought to be caused by propagation of a ring vortex formed at the ventricular base. Vortices are formed during the acceleration phases of the early and atrial filling waves. During the deceleration phases, the vortices are amplified and convected into the ventricle ( Figs. 11-3 and 11-4 ). The formation of a vortex is affected by the size of the inlet (mitral orifice) and the geometry and size of the receiving chamber. In small, tubular ventricles there is predominant columnar flow with no space from the mitral leaflets to the ventricular wall for vortex formation. In dilated ventricles with decreased ratio of mitral valve orifice to ventricular diameter, there would be predominant vortex formation and propagation. In this circumstance, flow velocities at the mitral tips can be relatively high, but the velocity of the front wave propagation remains slow. This explains why patients with a restrictive filling pattern can have a peak E-wave velocity greater than 1 m/sec at the level of the mitral level tips and at the same time a flow velocity propagation of 40 cm/sec or less. In in vitro modeling, the ratio of flow velocity to vortex propagation is around 2:1.


Obtaining and Measuring Flow Propagation
CMM flow propagation is usually measured from the apical four-chamber view. The M-mode cursor is carefully aligned with direction of flow, maximizing the distance from mitral tips to apex. At least 4 cm of CMM depth should be obtained and displayed at 100 cm/sec sweep speed. In the initial description by Brun, CMM flow propagation was measured as the slope of color-noncolor interface. The use of the slope of the color-noncolor interface is limited by its interference with isovolumic flow. Since then, several other methods have been proposed. This constitutes one of the main limitations of this technique, as it is difficult to compare studies among different authors. The slope of the first aliasing velocity has also been used by several authors, setting the aliasing velocity at a percentage (40%–70%) of the maximal inflow velocity (see Fig. 11-2 ). An aliasing velocity of 40% of the peak E-wave velocity appears to be more reproducible and to better reflect the velocity of propagation. There are no consensus guidelines as to whether phase I or phase II should be measured. Garcia et al. proposed the use of phase II when present.
Stugaard et al. used a computer algorithm to detect the maximal velocities along the center of the flow propagation wave and measured the time delay between the velocity at mitral tips and apex. This method is attractive, for it appears to be more objective, but it is not widely available. An automated way to measure the slope of Vp has been described. Normal values depend on the methodology used. With the slope of first aliasing (40%–50% of peak E velocity), values greater than 45 cm/sec are considered normal. The interobserver variability of Vp measurements has been reported to be around 12% but can be as high as 20%.
Late (A Wave) Color M-Mode Flow Propagation
Most of the studies have focused in the propagation of early mitral inflow. There are limited data about the importance and clinical significance of the propagation of flow during atrial contraction. There is a fundamental difference between early and late flow propagation. During early propagation, blood is pulled into the ventricle, while during late propagation, it is pushed (atrial contraction). A ratio of early to late flow propagation has been reported in patients with pseudonormal filling patterns, although the advantage of this over early Vp alone or E/Vp is unclear.
Color M-Mode Intraventricular Pressure Gradients
Given the complexity of CMM inflow patterns and the variability of the measurements of flow propagation, a more objective analysis of LV filling is desirable. It is well known that the presence of regional pressure differences in the left ventricle is related to LV relaxation and suction. Ling et al. demonstrated in a canine model the presence of regional diastolic IVPGs between the base and the apex of the left ventricle. These gradients resulted in the active filling of the ventricle. Courtois et al. validated these findings and later demonstrated a reduction in IVPG during myocardial ischemia in an animal model. These IVPGs are related to elastic recoil and to apical untwisting. Greenberg and Thomas used the Euler equation to calculate local pressure gradients,
∂ p ∂ s = – p [ ∂ v ∂ t + v ∂ v ∂ s ] ,
Δ P IV ( t ) = ∫ base apex ∂ p ∂ s d s
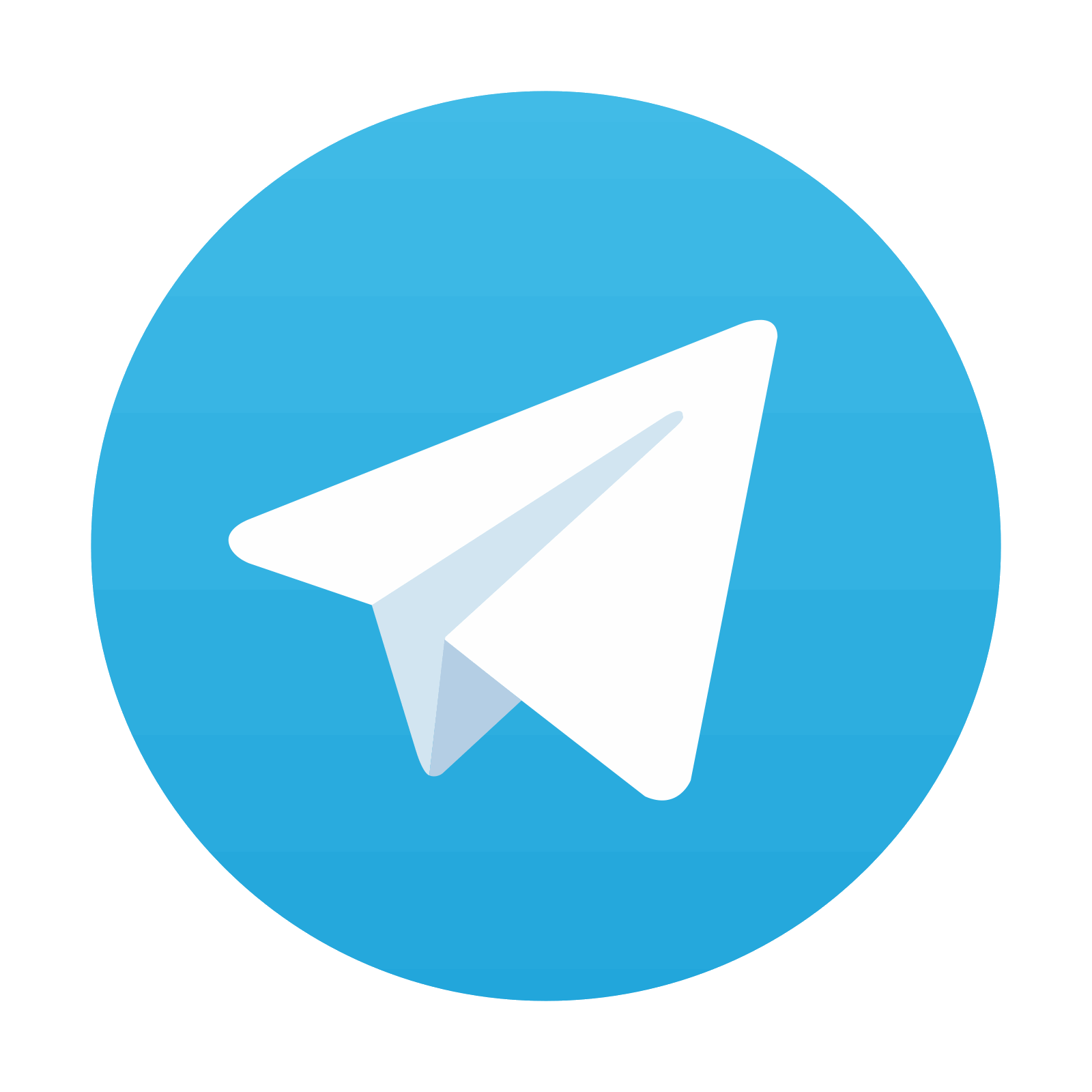
Stay updated, free articles. Join our Telegram channel
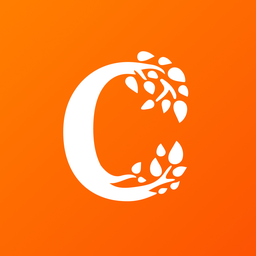
Full access? Get Clinical Tree
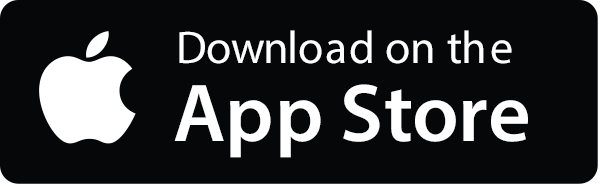
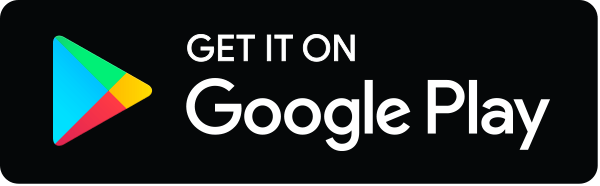