Abstract
During the 8-month period of gestation, the fetal lung is formed from a primordial mass of cells, arising as a small bud of foregut endoderm, which becomes an elaborately branched gas exchange unit with a delicately entwined vascular tree consisting of dozens of cell types. Failure to complete the task of lung morphogenesis often presents at the time of birth, when the newborn must adapt to airbreathing. In this chapter, the major events taking place during lung morphogenesis and differentiation are summarized. Dominant signaling and transcriptional pathways that direct the proliferation, migration, and differentiation of multiple cell types to form the adult lung are described and linked to congenital malformations and lung immaturity. Impaired lung formation and function often present as lung disorders in the neonatal period and infancy. The roles of coding and noncoding portions of the genome, including the epigenome, are integrated to coordinate gene expression during lung development, specifying cell identities and functions necessary for postnatal life. Throughout the chapter, we highlight how these developmental processes are relevant to congenital malformations and disorders of lung function, providing the framework for how the mature lung responds to injury and disease.
Keywords
lung, formation, differentiation, branching morphogenesis, congenital malformations, molecular and cell biology of lung development
Introduction
The adult human lung consists of a gas exchange area of approximately 100 m 2 that provides oxygen delivery and carbon dioxide removal required for cellular metabolism. In evolutionary terms, the lung represents a relatively late phylogenetic solution for the efficient gas exchange needed for terrestrial survival of organisms of increasing size, an observation that may account for the similarity of lung structure in vertebrates. The respiratory system consists of mechanical bellows and conducting tubules that bring inhaled gases to a large gas exchange surface that is highly vascularized. Alveolar epithelial cells (AECs) come into close apposition to pulmonary capillaries, providing efficient transport of gases from the alveolar space to the pulmonary circulation. The delivery of external gases to pulmonary tissue necessitates a complex organ system that (1) keeps the airway free of pathogens and debris, (2) maintains humidification of alveolar gases and precise hydration of the epithelial cell surface, (3) reduces collapsing forces inherent at air-liquid interfaces within the air spaces of the lung, and (4) supplies and regulates pulmonary blood flow to exchange oxygen and carbon dioxide efficiently. This chapter provides a framework for understanding the molecular mechanisms that lead to the formation of the mammalian lung, focusing attention to processes contributing to cell proliferation and differentiation involved in organogenesis and postnatal respiratory adaptation. Where possible, the pathogenesis of congenital or postnatal lung disease is considered in the context of the molecular determinants of pulmonary morphogenesis and function.
Organogenesis of the Lung
Formation of the Basic Body Plan
Events critical to organogenesis of the lung begin with formation of anterior-posterior (A-P), dorsal-ventral, and left-right axes in the early embryo, which, in turn, specifies the basic body plan of each organism. The formation of these axes is determined by genes that control cellular proliferation and differentiation and depends on complex interactions among many cell types. The fundamental principles determining embryonic organization have been elucidated in simpler model organisms (e.g., amphibians, fruit flies, sea urchins, snails, worms, and zebra fish) and applied to increasingly complex organisms (e.g., mouse and human), as the genes determining axial segmentation, organ formation, cellular proliferation and differentiation have been identified. Segmentation and organ formation in the embryo are profoundly influenced by sets of master control genes that include various classes of transcription factors. Critical to formation of the axial body plan are the homeotic, or HOX, genes. HOX genes are arrayed in clearly defined spatial patterns within clusters on several chromosomes. HOX gene expression in the developing embryo is determined in part by the position of the individual genes within these gene clusters, which are aligned along the chromosome in the same order as they are expressed along the A–P axis. Complex organisms have more individual HOX genes within each locus and have more HOX gene loci than simpler organisms. In addition, HOX genes encode nuclear proteins that bind to DNA via a highly conserved homeodomain motif that modulates the transcription of specific sets of target genes. The temporal and spatial expression of these nuclear transcription factors, in turn, control the expression of other HOX genes and their transcriptional targets during morphogenesis and cytodifferentiation. Expression of HOX genes influences many downstream genes, such as transcription factors, growth factors, signaling peptides, and cell adhesion molecules, which are critical to the formation of the primitive endoderm from which the respiratory epithelium is derived.
Specification of the Foregut Endoderm
The primitive endoderm develops very early in the process of embryogenesis, that is, during gastrulation and prior to formation of the intraembryonic mesoderm, ectoderm, and the notochord, which occurs in humans at 3 weeks postconception (WPC). Specification of the definitive endoderm and the primitive foregut requires the activity of a number of nuclear transcription factors that regulate gene expression in the embryo, including forkhead box A2 (FOXA2) (also known as hepatocyte nuclear factor 3-beta, or HNF-3β), GATA-binding protein 6 (GATA6), sex-determining region Y ( S RY)-related high mobility group (HMG)-b ox (SOX) 17 (SOX17), SOX2, β-catenin, retinoic acid receptors (RAR), and members of the T-box family of transcription factors. Genetic ablation of these transcription factors disrupts formation of the primitive foregut endoderm and its developmental derivatives, including the trachea and the lung. Some of these transcription factors are also expressed in the respiratory epithelium later in development when they play important roles in the regulation of cell differentiation and organ function.
Lung Morphogenesis
Lung morphogenesis is initiated during the embryonic period of fetal development (3–4 WPC in the human) with the formation of a small saccular outgrowth of the ventral wall of the foregut endoderm, a process that is induced by expression of WNT2/2b and fibroblast growth factor (FGF) 10, in the adjacent splanchnic mesoderm ( Fig. 2.1 ). After the main bronchial tubes form the lung tubules, they undergo branching morphogenesis to create the highly arborized, stereotypical patterns of airway segmentations. This region of the ventral foregut endoderm is delineated by epithelial cells expressing the homeobox gene, NKX2-1 (also known as thyroid transcription factor-1 [TTF1]), which is the earliest known marker of the prospective respiratory epithelium. Thereafter, lung development can be subdivided into five distinct periods of morphogenesis based on the morphologic characteristics of the tissue ( Table 2.1 ; Fig. 2.2 ). While the timing of this process is highly species specific, the anatomic events underlying lung morphogenesis are shared by all mammalian species. Details of human lung development are described in the following sections, as well as in several published reviews.

Period | Age (WPC) | Structural Events |
---|---|---|
Embryonic | 3–6 | Lung buds; trachea, main stem, lobar, and segmental bronchi; trachea and esophagus separate |
Pseudoglandular | 6–17 | Subsegmental bronchi, terminal bronchioles, and acinar tubules; mucous glands, cartilage, and smooth muscle |
Canicular | 16–26 | Respiratory bronchioles, acinus formation and vascularization; type I and II AEC differentiation |
Saccular | 26–36 | Dilation and subdivision of alveolar saccules, increase of gas-exchange surface area, and surfactant synthesis |
Alveolar | 36—maturity | Further growth and alveolarization of lung; increase of gas-exchange area and maturation of alveolar capillary network; increased surfactant synthesis |

The Embryonic Period (3–7 Weeks Postconception)
Relatively undifferentiated epithelial cells of the primitive foregut endoderm form tubules that invade the surrounding splanchnic mesoderm and undergo branching morphogenesis. This process requires highly controlled epithelial cell proliferation and migration to direct dichotomous branching of the respiratory tubules, which form the main-stem, lobar, and segmental bronchi of the primitive lung (see Table 2.1 ; Fig. 2.2 ). The respiratory epithelium remains relatively undifferentiated and is lined by columnar epithelium during this process. Proximally, the trachea and esophagus also separate into two distinct structures during this period. Experimental removal of mesenchymal tissue from the embryonic endoderm at this time arrests branching morphogenesis, demonstrating the critical role of mesenchyme in the formation of the respiratory tract. Interactions between epithelial and mesenchymal cells are mediated by a variety of signaling pathways that involve the binding of secreted peptides and extracellular matrix proteins to their respective cell surface receptors, which regulates gene transcription in differentiating lung cells. These epithelial-mesenchymal interactions involve both autocrine and paracrine signaling pathways that are critical for lung morphogenesis ( Fig. 2.3 ). Paracrine signaling pathways important for initial formation of the lung bud, as well as expansion and branching of the primitive respiratory tubules, include (1) FGF10/FGFR2, (2) sonic hedgehog (SHH/PTCH1), (3) transforming growth factor-beta (TGFβ/TGFβR2), (4) bone morphogenic protein B (BMP4/BMPR1b), (5) retinoic acid (RA/RARα, β, γ), (6) WNT (WNT2/2b, 7b, 5a and R-spondin with their receptors Frizzled and LRP5/6), and (7) the β-catenin signaling pathways. Nuclear transcription factors that are active in the primitive respiratory epithelium during this period include NKX2-1, FOXA2, GATA6, and SOX2. Likewise, transcription factors active in the mesenchyme at this time include (1) the HOX family of transcription factors (HOXA5, B3, B4); (2) the SMAD family of transcription factors (SMAD2, 3, 4), downstream transducers of the TGFβ/BMP signaling pathway; (3) the LEF/TCF family of transcription factors, downstream transducers of β-catenin, (4) the GLI-KRUPPEL family of transcription factors (GLI1, 2, 3), downstream transducers of SHH signaling; (5) the hedgehog-interacting protein, HHIP1, that binds SHH; and (6) FOXF1, another SHH target. Disruption of many of these transcription factors and signaling pathways in experimental animals causes impaired morphogenesis, resulting in laryngotracheal malformations, tracheoesophageal fistulas, esophageal and tracheal stenosis, esophageal atresia, defects in pulmonary lobe formation, pulmonary hypoplasia, or pulmonary ageneisis.

Although formation of the larger, more proximal, conducting airways, including segmental and subsegmental bronchi, is completed by 6 WPC, both epithelial and mesenchymal cells of the embryonic lung remain relatively undifferentiated. At this stage, trachea and bronchial tubules lack underlying cartilage, smooth muscle, or nerves; and the pulmonary and bronchial vessels are not well developed. Vascular connections with the right and left atria are established by a common cardiopulmonary vascular progenitor at the end of this period (6–7 WPC), creating the primitive pulmonary vascular bed. Human developmental anomalies occurring during this period of morphogenesis include laryngeal, tracheal, esophageal, and bronchial atresia; tracheoesophageal and bronchoesophageal fistulas; tracheal and bronchial stenosis; bronchogenic cysts; ectopic lobes; extrapulmonary sequestration; and pulmonary agenesis. Some of these congenital anomalies are associated with documented mutations in the genes involved in early lung development, such as GLI3 (tracheoesophageal fistula found in Pallister-Hall syndrome), FGFR2 (various laryngeal, esophageal, tracheal, and pulmonary anomalies found in Pfeiffer, Apert, or Crouzon syndromes), and SOX2 (esophageal atresia and tracheoesophageal fistula found in anophthalmia-esophageal-genital, or AEG, syndrome).
The Pseudoglandular Period (6–17 Weeks Postconception)
The pseudoglandular stage is so named because of the distinct glandular appearance of the lung from 6 to 17 WPC. During this period, the lung consists primarily of epithelial tubules surrounded by a relatively thick mesenchyme. Branching of the airways continues with formation of the terminal bronchioles and primitive acinar structures, which is completed by the end of this period (see Table 2.1 ; Fig. 2.2 ). During the pseudoglandular period, epithelial cell differentiation is increasingly apparent; and deposition of cellular glycogen and expression of genes expressed selectively in the distal respiratory epithelium are initiated. The surfactant proteins, SFTPB and SFTPC, are first detected in primitive type II AECs at 12–14 weeks of gestation. Tracheobronchial glands begin to form in the proximal conducting airways; and the airway epithelium is increasingly complex, with basal, mucous, ciliated, and nonciliated secretory cells being detected. Neuroendocrine cells, often forming clusters of cells, termed neuroepithelial bodies , express a variety of neuropeptides and transmitters (e.g., bombesin, calcitonin-related peptide, serotonin, and others) and are increasingly apparent along the bronchial and bronchiolar epithelium. Smooth muscle and cartilage are now observed adjacent to the conducting airways, and the pulmonary vascular system develops in close relationship to the bronchial and bronchiolar tubules between 9 and 12 WPC when smooth muscle actin and myosin can be detected in these vascular structures.
During this period, FGF10, BMP4, TGFβ, β-catenin, and the WNT signaling pathways continue to be important for branching morphogenesis, along with several other signaling peptides and growth factors, including members of the (1) FGF family (FGF1, FGF2, FGF7, FGF9, FGF18); (2) TGFβ family, such as the SPROUTYs (SPRY2, SPRY4), which antagonizes and limits FGF10 signaling, and LEFTY/NODAL, which regulates left-right patterning; (3) epithelial growth factor (EGF) and transforming growth factor alpha (TGFα) family, which stimulate cell proliferation and cytodifferentiation; (4) insulin growth factor (IGFI, IGFII) family, which facilitates signaling of other growth factors; (5) platelet derived growth factor (PDGFA, PDGFB) family, whose members are mitogens and chemoattractants for mesenchymal cells; and (6) vascular endothelial growth factor (VEGFA, VEGFC) family, which regulates vascular and lymphatic growth and patterning. Many of the nuclear transcription factors that were active during the embryonic period of morphogenesis continue to be important for lung development. Additional transcriptional factors important for specification and differentiation of the primitive lymphatic vessels in the mesenchyme at this time include (1) SOX18, (2) the paired-related homeobox gene, PRX1, (3) the divergent homeobox gene, HEX, and (4) the homeobox gene, PROX1.
A variety of congenital defects may arise during the pseudoglandular stage of lung development, including tracheomalacia and bronchomalacia, intralobar bronchopulmonary sequestration, congenital pulmonary airway malformations (formerly cystic adenomatoid malformations), acinar aplasia or dysplasia, alveolar capillary dysplasia with or without misalignment of the pulmonary veins, congenital pulmonary lymphangiectasia, and other pulmonary vascular malformations. The pleuroperitoneal cavity also closes early in the pseudoglandular period. Failure to close the pleural cavity, often accompanied by herniation of the abdominal contents into the chest (congenital diaphragmatic hernia), may cause pulmonary hypoplasia.
The Canalicular Period (16–26 Weeks Postconception)
The canalicular period is characterized by further growth and subdivision of the acinar tubules, rapid expansion of the pulmonary capillary bed, thinning of the pulmonary mesenchyme, and formation of the alveolar-capillary membrane (see Table 2.1 ; Fig. 2.2 ). By the end of this period, the terminal bronchioles have divided to form two or more respiratory bronchioles, and each of these has divided into multiple acinar tubules, forming the primitive pulmonary acini. Epithelial cell differentiation becomes increasingly complex and is especially apparent in the distal regions of the lung parenchyma. Bronchiolar cells express differentiated features, such as cilia, and secretory cells synthesize cell-specific secretory proteins, such as mucin and secretoglobin 1A1 (SCGB1A1). Cells lining the distal tubules assume cuboidal shapes and express increasing amounts of surfactant phospholipids and the associated surfactant proteins, surfactant protein A (SFTPA), SFTPB, and SFTPC. Lamellar bodies, composed of surfactant phospholipids and proteins, are seen in association with rich glycogen stores in the cuboidal pretype II AECs that line the distal acinar tubules. Some cells of the acinar tubules become squamous, acquiring features of typical type I AECs. Capillaries surround the distal acinar tubules and establish contact with the adjacent epithelium to form the primitive alveolar-capillary membrane, which ultimately forms the gas exchange region of the mature lung. By the end of the canalicular period in the human infant (26–28 WPC), gas exchange can be supported after birth, especially when surfactant is provided by administration of exogenous surfactant. Surfactant synthesis and parenchymal thinning can be accelerated by glucocorticoids at this time, which is why they are administered to mothers to prevent respiratory distress syndrome (RDS) after premature birth. Abnormalities of lung development that occur during the canalicular period include congenital alveolar dysplasia, alveolar capillary dysplasia, and pulmonary hypoplasia, and the latter is caused by (1) diaphragmatic hernia, (2) compression due to thoracic or abdominal masses, (3) prolonged rupture of membranes causing oligohydramnios, or (5) renal agenesis, in which amniotic fluid production is impaired. While postnatal gas exchange can be supported late in the canalicular stage, infants born during this period generally suffer severe complications related to decreased pulmonary surfactant, which causes RDS and bronchopulmonary dysplasia, and the latter is a complication secondary to the intensive care therapy and increasing survival of very preterm infants.
The Saccular (26–36 Weeks Postconception) and Alveolar Periods (36 Weeks Postconception Through Adolescence)
These periods of lung development are characterized by increased thinning of the respiratory epithelium and pulmonary mesenchyme, further growth and expansion of the pulmonary acini, and development of the distal alveolar capillary network (see Table 2.1 ; Fig. 2.2 ). Maturation of type II AECs occurs in association with increased synthesis of surfactant phospholipids, the surfactant proteins, SFTPA, SFTPB, and SFTPC, and the adenosine triphosphate (ATP)-binding cassette transporter, ABCA3, a phospholipid transporter important for lamellar body biogenesis. Squamous type I AECs line an ever-increasing proportion of the surface area of the distal lung. Adjacent interstitial capillaries become closely associated with the squamous type I AECs, decreasing the diffusion distance for oxygen and carbon dioxide between the alveolar space and capillary bed. Basal laminae of the epithelium and endothelium fuse to form the thin-walled alveolar-capillary membrane; and the interstitial tissue contains increasing amounts of extracellular matrix, including elastin and collagen. Secondary alveolar septa form, which partition the terminal saccules into true alveoli, greatly increasing the surface area of the lung available for gas exchange. In the human lung, the alveolar period begins near the time of birth and continues through the first decade of life, during which time, the lung grows primarily by septation, proliferation, and thinning of the alveolar walls, as well as by elongation and luminal enlargement of the conducting airways. Pulmonary arteries enlarge and elongate in close relationship to the increased growth of the lung. Pulmonary vascular resistance decreases, and considerable remodeling of the pulmonary vasculature and capillary bed continue during the postnatal period. Lung growth remains active until early adolescence, when the entire complement of approximately 300 million alveoli has been formed.
Signaling pathways that are critical for growth, differentiation, and maturation of the alveolar epithelium and capillary bed during these periods include the FGF, platelet-derived growth factor (PDGF), vascular endothelial growth factor (VGEF), RA, BMP, WNT, β-catenin, and NOTCH signaling pathways. For example, targeted deletion of the FGF receptors, Fgfr3 and Fgfr4, blocks alveologenesis in mice; likewise, targeted deletion of Pdgfa interferes with myofibroblast proliferation and migration, resulting in failure of alveologenesis and postnatal alveolar simplification in mice. Notch2, expressed by type II AECs in the developing lung, induces PDGFA expression, activating platelet-derived growth factor receptor (PDGFR) signaling in alveolar myofibroblast progenitors, which is required for myofibroblast differentiation and normal alveologenesis. Recently, a WNT responsive epithelial cell has been identified that serves as a progenitor for both type I and type II AECs during perinatal alveologenesis.
Nuclear transcription factors found earlier in lung development, that is, FOXA2, NKX2-1, GATA6 and SOX2, continue to be important for maturation of the lung, influencing sacculation, alveolarization, vascularization, and cytodifferentiation of the peripheral lung. Transcription factors associated with cytodifferentiation during these periods include (1) FOXJ1 (ciliated cells), (2) MASH1 (or HASH1) and HES1 (neuroendocrine cells), (3) FOXA3 and SPDEF (mucus cells), (4) ETV5/ERM (type II AECs), and (5) HOPX (type I AECs). Morphogenesis and cytodifferentiation are further influenced by additional transcription factors expressed in the developing respiratory epithelium at this time, including (1) several ETS factors (ETV5/ERM, SPDEF, ELF3/5); (2) SOX genes (SOX-9, SOX11, SOX17); (3) nuclear factor of activated T cells/calcineurin-dependent signaling (NFATC); (4) nuclear factor-1 (NF-1); (5) CCAAT/enhancer binding protein alpha (CEBPα); (6) Krüppel-like factor 5 (KLF5); (7) transcription factors, GLI2/GLI3, SMAD3, FOXF1, POD1, and HOX (HOXA5, HOXB2-B5); all of which are expressed in the mesenchyme.
Molecular Mechanisms Directing Lung Development
Remarkable advances in our understanding of gene regulatory mechanisms are providing an evermore detailed understanding of the processes by which the genetic code is translated into specific cell types and their organization in organs and tissues. Numerous molecular regulatory mechanisms exist to precisely coordinate cell proliferation, lineage commitment, and subsequent terminal differentiation of over 40 distinct, but closely intertwined, cell types that form the mature mammalian lung. Although the “blueprints” for organogenesis are encoded in the organism’s genomic DNA, this information must be transmitted to cells of the developing lung by transcribing regions of DNA into messenger RNA (mRNA) and then by translating the mRNAs into protein. These cellular proteins, in turn, influence morphologic, metabolic, and proliferative behaviors of cells throughout development. Points of regulation include control over transcriptional RNA expression, mRNA stability, protein translation, and degradation, as well as further refinement in the abundance of mRNAs and proteins synthesized by each specific cell, which ultimately determine its structure and function. At each step in this cascade of information, numerous regulatory mechanisms exist, and many of which are highly interactive with complex feedback and feed-forward loops, as well as built-in redundancies, to assure precise specification of normal developmental patterns ( Fig. 2.4 ).

Transcriptional Mechanisms Regulating Gene Expression
Transcription Factors and Gene Regulatory Networks
Transcription factors, which are nuclear proteins that bind to specific DNA nucleotide sequences (motifs) in regulatory regions throughout the genome, represent one major mode of transcriptional regulation. The binding of transcriptional factors to the cis-acting elements influences the activity of RNA polymerase II, which binds, in turn, to sequences near the transcription start site of target genes, initiating mRNA synthesis. Transcription factors may recruit additional proteins to the site of binding, modifying various chemical signatures on the DNA or chromatin (e.g., methylation, acetylation) that can continue to influence gene transcription long after the transcription factor itself leaves the region. Numerous families of transcription factors have been identified, and their activities are regulated by a variety of mechanisms, including posttranslational modification and interactions with other proteins or DNA, as well as by their ability to translocate or remain in the nucleus. Due to the large number of individual transcription factors and transcription-binding sites across the genome, these proteins interact combinatorally to form complex gene regulatory networks that direct patterns of gene transcription throughout development.
Although our knowledge of the various factors regulating gene transcription during lung development is still in its infancy, a number of transcription factors and signaling networks that play critical roles in lung morphogenesis have been identified. A general schematic of important transcriptional regulators of lung epithelial morphogenesis is seen in Figs. 2.5 and 2.6 . Lung morphogenesis depends on formation of definitive endoderm, which, in turn, signals from the splanchnic mesenchyme to initiate organogenesis along the foregut, forming thyroid, liver, pancreas, lung, and portions of the gastrointestinal tract. FOXA2, a member of the winged helix family of transcription factors, is a “pioneering” transcription factor that is known to play a critical role in committing progenitor cells of the endoderm to form the primitive foregut. Global loss of Foxa2 in mice results in catastrophic failure of development at the time of gastrulation. Loss of Foxa2 at later developmental timepoints, alone or in combination with the related transcription factor Foxa1, has revealed its essential role in specification of the pancreas and liver and formation of the lung epithelium, and it influences the expression of specific genes in the respiratory epithelium later in development. Conditional deletion of Foxa2 in the lung epithelium of mice highlights its ongoing role in both the proximal and distal lung epithelium. Conditional loss of Foxa2 prior to birth resulted in impaired maturation of type II cells, altered regulation of surfactant protein and phospholipid production, and death from RDS after birth. Loss of Foxa2 after birth caused goblet cell metaplasia, airspace enlargement, and inflammation during the postnatal period. Thus, FOXA2 plays a critical role in specification of foregut endoderm in the early embryo, and it is used again in the perinatal and postnatal period to direct surfactant production, alveolarization, postnatal lung function, and homeostasis (see Fig. 2.5 ).


The transcription factor NXK2-1 is the earliest known marker of lung formation. This 38-kd nuclear protein contains a homeodomain DNA-binding motif, and it is critical for formation of the lung and for regulation of a number of highly specific gene products produced selectively in the respiratory epithelium. NKX2-1 is also expressed in the thyroid and in specific regions of the developing central nervous system. Ablation of Nkx2-1 in mice impairs lung morphogenesis, resulting in hypoplastic lungs lined by a poorly differentiated respiratory epithelium and lacking gas exchange areas. Substitution of a mutant Nkx2-1 gene that lacks phosphorylation sites substantially rescues lung function in the Nkx2-1 knockout mouse. Nkx2-1 directly regulates the transcription of numerous downstream targets, including genes involved in surfactant homeostasis, fluid and electrolyte transport, host defense, and vasculogenesis. Just as important, NKX2-1 functions in concert with other transcription factors, including GATA6, the NF-1 family of transcription factors, STAT3, NFAT, FOXA1/FOXA2, and RARs to form a tightly integrated gene regulatory network to direct lung epithelial development and differentiation. For example, NKX2-1 gene transcription itself is modulated by the activity of FOXA2, which binds to the promoter enhancer region of the NKX2-1 gene, thus creating a transcriptional network. The stoichiometry, timing, and distinct combinations of transcription factors, as well as posttranscriptional modification of these transcription factors, are all critical factors in determining the precise transcriptional output at each stage of development (see Fig. 2.5 ), and our comprehensive understanding of how these signals are integrated on the systems-level is just beginning to emerge.
Cis-Regulatory Networks Controlling Gene Expression
Since the launch of the ENCODE (Encyclopedia of DNA elements) project, it has become increasingly clear that a significant fraction of the genome outside of protein-coding genes plays a key role in regulating gene expression. Cis-regulatory elements are a key class of regulatory noncoding DNA sequences, which act to regulate the transcription of a neighboring gene (although the distance of the regulated gene may be many megabases away). Promoters and enhancers are examples of cis-regulatory elements and characteristically contain many transcription factor binding motifs, integrating signals derived from the intricate gene regulatory networks described above. Promoter elements are essential for proper assembly of the key machinery necessary to initiate and establish transcription. Enhancers are “canonically defined as short (~100–1000 bp) noncoding DNA sequences that act to drive transcription independent of their relative distance, location, or orientation to their cognate promoter.” Consisting of clusters of transcription factor motifs, combinations of transcription factors interact with enhancer elements, recruit additional cofactor protein complexes, and act combinatorally to interact with the promoter of their regulated gene to modulate gene expression. Genome-wide association studies (GWAS) of many common disorders, including lung diseases such as interstitial pulmonary fibrosis, chronic obstructive pulmonary disease, and asthma, demonstrate that many of the genetic variants identified to date, which have been implicated in susceptibility to these diseases, fall within these noncoding regions of the genome. Thus, even though our understanding of the location and function of all cis-regulatory elements (referred to as the “cistrome”) critical for proper pulmonary development and function remains limited, a deeper understanding of this area of gene regulation will be essential to make progress in understanding the pathogenesis of a wide variety of common pulmonary disorders.
Epigenetic Mechanisms: DNA Methylation and Chromatin State
Genomic DNA does not exist in isolation within the cell, but genomic DNA instead is wrapped around proteins called histones in a very orderly fashion that consists of multiple levels of coiling and packing. Both the efficiency or “tightness” with which chromatin is compacted, as well as numerous associated chemical modifications to either the histone proteins or the DNA itself, are emerging as critical mechanisms by which gene expression is regulated during lung development. Methylation of cytosine at position 5 of the pyrimidine ring (largely on cytosines found in the CpG context) is the best studied of these “epigenetic” modifications, with roles in a large variety of biological processes, including X-chromosome inactivation, genomic imprinting, repression of transposable elements, aging, and cancer. In the context of regulating gene expression, methylation of a promoter region generally acts to repress expression of that gene. The promoters of active genes are typically unmethylated regions, or UMRs, whereas distal regulatory elements such as enhancers often have low levels of methylation, or LMRs. These methylation states have been observed to change dynamically during embryonic stem cell models of development and in organ systems such as the brain. Moreover, widespread differences in methylation are observed in a variety of lung diseases such as interstitial pulmonary fibrosis. The precise mechanisms, however, by which DNA methylation directs normal development and subsequent homeostasis of the adult lung remain poorly understood. Over 40 posttranslational modifications to the peptide tails of histones have been described, and they are associated with at least a dozen different chromatin “states.” For example, tri-methylation of lysine 4 on histone H3 (notated as H3K4me ) is found on active promoter regions, whereas colocalization of H3K4me and H3K27ac mark active enhancers. Other marks, such as H3K27me , are found on regions of silenced heterochromatin. Large-scale efforts such as the ENCODE Project and International Human Epigenome Consortium (IHEC) have generated thousands of datasets to create maps of the cis-regulatory landscape in dozens of tissue- and cell-types. It is thought that these various chemical marks act to integrate and retain the directions provided by the binding of transcription factors to chromatin throughout development, helping to “lock-in” specific cell fate decisions and provide cellular memory for these transient, but powerful, developmental cues long after they have passed. Dysregulation of chromatin state has recently been recognized to contribute to a wide variety of human disorders from congenital heart disease to lung adenocarcinoma. However, whether these posttranslational modifications of histones are merely associated with various regulatory states or play direct and causal roles in regulation of gene expression is just beginning to be explored, particularly in the context of lung development.
Epigenetic Mechanisms: Chromatin Topology/3D Structure
It has long been recognized that many cis-regulatory elements such as enhancers act at great distances, often thousands or even millions of bases-pairs, to regulate the expression of their target genes. Three-dimensional folding of chromatin is essential to mediate these interactions, bringing distant enhancer elements with bound transcription factors and coactivator protein complexes into physical proximity to their target gene promoters. Chromatin conformation capture (3C)-based technologies have revealed that the genome is organized into discrete physical domains, called topological-associated domains, or TADs, often forming loops of coregulated genes. Other regions of chromatin have been observed to associate with the nuclear membrane (laminin-associated domains or LADs), which typically consist of genes that are silenced or only expressed at low levels, that are thought to help further partition chromatin within the nucleus. Examples of local or global dysregulation of chromatin topology have been reported in human diseases, ranging from blood diseases to Down syndrome, and forced chromatin looping has even been used as a treatment strategy for hemoglobinopathies. Future research in this area is likely to have significant implications for our understanding of lung development and pulmonary diseases.
Nontranscriptional Mechanisms Regulating Morphogenesis
Noncoding RNA
With the advent of widespread availability of next-generation sequencing-based analysis of RNA, it has become clear that many regions of the genome (as much as 60%–80%) are transcribed into RNA, even if many of these RNAs do not progress to make a protein. The transcripts are called “non-coding RNAs” (ncRNA) and are typically divided into two broad categories based on their size: transcripts greater than 200 bp are referred to as “long non-coding RNAs” (lncRNAs), and their smaller counterparts (<200 bp) are referred to as small ncRNAs. A wide variety of small ncRNAs have been described, including microRNAs (miRNAs), small nuclear RNAs (snRNAs), and Piwi-interacting RNAs (piRNAs). A number of miRNAs have been specifically implicated in lung development, including miR-17-92, miR20a, miR-302-367, miR106a-106b, and miR-34/449. For example, loss of the miR-17-92 cluster leads to pulmonary hypoplasia due to decreased cellular proliferation, while overexpression enhances proliferation and differentiation of the lung epithelium. More broadly, loss of the Dicer complex, a key component of the machinery necessary to process longer, immature RNA transcripts (pri- or pre-miRNAs) into their mature miRNA form, results in severe defects in branching morphogenesis, and germline mutations in the DICER gene have been identified in familial cases of the rare pediatric lung malignancy pleuropulmonary blastoma. Long noncoding RNAs also exhibit dynamic expression patterns throughout development, and new studies have started to unravel important contributions to lung organogenesis. An important subset of lncRNAs appears to be genomically positioned near critical transcription factors, including Nkx2-1, Foxa2, and Foxf1. Loss of the lncRNA, Nanci, adjacent to the transcription factor, Nkx2-1, results in a reduction in NKX2-1 expression and recapitulates aspects of the Nkx2-1 haploinsufficiency phenotype, while loss of the lncRNA, Fendrr, adjacent to Foxf1 results in a phenotype similar to the human lung developmental disorder alveolar capillary dysplasia. Other lncRNAs, such as MALAT1, have been implicated in the pathogenesis of multiple types of malignancy, including lung cancer, but global loss of MALAT1 demonstrated that this lncRNA is not necessary for normal pulmonary development. Although individual elements may be dispensable for normal development, it is likely that lncRNAs, similar to miRNAs and many of the other gene regulatory mechanisms discussed earlier, act in highly redundant, coordinated systems to fine-tune gene expression throughout life and will play key roles in response to physiologic stress and disease.
Receptor-Mediated Signal Transduction
Receptor-mediated signaling is well recognized as a fundamental mechanism for transducing extracellular information. Such signals are initiated by the occupancy of membrane-associated receptors capable of transducing additional signals (known as secondary messengers), such as cyclic adenosine monophosphate, calcium, and inositide phosphates, which influence the activity and function of intracellular proteins (e.g., kinases, phosphatases, and proteases). These proteins, in turn, may alter the abundance of transcription factors, the activity of ion channels, or changes in membrane permeability, which subsequently modify cellular behaviors. Receptor-mediated signal transduction, induced by ligand-receptor binding, mediates endocrine, paracrine, and autocrine interactions on which cell differentiation and organogenesis depend. For example, signaling peptides and their receptors, such as FGF, SHH, WNT, BMP, VEGF, PDGF, and NOTCH have been implicated in organogenesis of many organs, including the lung.
Gradients of Signaling Molecules and Localization of Receptor Molecules
Chemical gradients within tissues, and their interactions with membrane receptors located at distinct sites within the organ, can provide critical information during organogenesis. Polarized cells have basal, lateral, and apical surfaces with distinct subsets of signaling molecules (receptors) that allow the cell to respond in unique ways to focal concentrations of regulatory molecules. Secreted ligands (e.g., FGFs, TGFβ/BMPs, WNTs, SHH, and HHIP1) are further influenced by binding of the ligand to basement membranes or to proteoglycans in the extracellular matrix. Spatial information is established by gradients of these signaling molecules and by the presence and abundance of receptors at specific cellular sites. Such systems provide positional information to the cell, which influences its behavior (e.g., shape, movement, proliferation, differentiation, and polarized transport).
Epithelial-Mesenchymal Interactions and Lung Morphogenesis
Branching morphogenesis, growth, and differentiation of the respiratory tract depends on precise reciprocal signaling between endodermally derived cells of the lung buds and the surrounding pulmonary mesenchyme. This interdependency depends on autocrine and paracrine interactions that are mediated by a diversity of signaling systems governing cellular behavior. Similarly, autocrine and paracrine interactions are known to be involved in cellular responses of the postnatal lung, generating signals that regulate cell proliferation and differentiation necessary for its repair and remodeling following injury. The splanchnic mesenchyme produces a number of signaling peptides critical for migration and proliferation of cells in the lung buds, including FGF10, FGF7, FGF9, BMP5, and WNT 2/2b, which activate receptors found on epithelial cells. In a complementary manner, epithelial cells produce WNT7b, WNT5a, SHH, BMP4, FGF9, VEGF, and PDGF that activate receptors and signaling pathways on target cells in the mesenchyme (see Fig. 2.3 ).
Branching Morphogenesis, Vascularization, and Alveologenesis
Three distinct processes, branching, vascularization, and alveologenesis are critical to morphogenesis of the mammalian lung. The major branches of the conducting airways of the human lung are completed by 16 WPC by a process of dichotomous branching, initiated by the bifurcation of the main-stem bronchi early in the embryonic period of lung development. Epithelial-lined tubules of ever-decreasing diameter are formed from the proximal to distal region of the developing lung. The terminal bronchioles divide into two or more respiratory bronchioles, which subdivide further into clusters of acinar tubules and buds at the periphery of the lung. Lung alveologenesis begins in the late canalicular period (16 WPC and thereafter) with expansion of the distal acinar tubules into terminal saccules. Further subdivision of these saccules results in the formation of the adult alveolar ducts and alveoli. During sacculation, a unique pattern of vascular supply forms the capillary network surrounding each terminal saccule, providing an ever-expanding gas exchange area that is completed in adolescence. Both vasculogenesis and angiogenesis contribute to formation of the pulmonary vascular system.
The gas-exchange regions of the lung are supplied by the pulmonary arterial system, which originates from the sixth pair of aortic arches. The pulmonary arteries extend into the surrounding mesoderm, where they accompany the developing airways, segmenting with each bronchial subdivision. Ultimately, these arteries anastomose with the pulmonary vascular plexus that is developing in the pulmonary mesenchyme around the peripheral acinar tubules. In contrast, the bronchial vasculature arises from the descending aorta, providing nutrient supply to the conducting airways. The pulmonary veins arise from the left atrium and divide several times before connecting to the pulmonary vascular plexus in the acinar parenchyma, completing the pulmonary circulation. In the adult, these veins are located primarily in the interlobular connective tissue septa that surround each pulmonary lobule. Lymphatic vessels form around the bronchi and pulmonary vessels, extending into the interlobular septa and the pleura. Parasympathetic and sympathetic nerve fibers form along the conducting airways and major vascular structures, extending as far as the alveolar ducts. Signaling via SHH, VEGFA, FOXF1, NOTCH, Ephrins, and PDGF plays a significant role in pulmonary vascular development. For example, VEGFA and its receptors (VEGFR1, VEGFR2) are critical factors for vasculogenesis in many tissues. Targeted inactivation of Vegf and Vefgfr1 in mice results in impaired angiogenesis, while overexpression of the VEGFA 164 isoform disrupts pulmonary vascular endothelium in newborn conditional transgenic mice, causing pulmonary hemorrhage. PROX1, a homeodomain transcription factor, is induced in a subset of venous endothelial cells during development and upregulates other lymphatic-specific genes, such as VEGFR3 and LYVE1, which are critical for development of the lymphatic network in the lung. Growth factors important for lymphatic development include VEGFC and its receptor, VEGFR3, as well as the angiopoietins, ANG1 and ANG2, and their receptors, TIE1 and TIE2. Insufficiency or targeted deletion of these factors in mice impairs lymphatic vessel formation.
Control of Lung Proliferation During Branching Morphogenesis
Both in vitro and in vivo experiments strongly support the concept that the mesenchyme produces signaling peptides and growth factors critical to the formation of respiratory tubules. Dissection of the splanchnic mesenchyme from the lung buds arrests cell proliferation, branching, and differentiation of the pulmonary tubules in vitro. Lung growth is influenced by mechanical factors, including the size of the thoracic cavity and by stretch. For example, complete occlusion of the fetal trachea in utero enhances lung growth, while drainage of lung liquid or amniotic fluid causes pulmonary hypoplasia. Regional control of proliferation is required for the process of branching morphogenesis: division is enhanced at the lateral edges of the growing bud and inhibited at branch points. Precise positional control of cell division is determined by polypeptides derived from the mesenchyme (e.g., growth factors or extracellular matrix molecules) that selectively decrease proliferation at clefts and increase cell proliferation at the edges of the bud. Proliferation in the respiratory tubule is dependent on a number of growth factors, including the FGF family of polypeptides. In vitro, FGF1, and FGF7 partially replace the requirement of pulmonary mesenchyme for continued epithelial cell proliferation and budding. FGF10 produced by the mesenchyme during lung development binds to and activates a splice variant of FGFR2 (FGFR2IIIb) that is present on respiratory epithelial cells, completing a paracrine loop. Blockade of FGFR2 signaling in the epithelium of the developing lung bud in vivo, using a dominant-negative FGF receptor mutant, completely blocked dichotomous branching of all conducting airway segments except the primary bronchi in mice. FGF10 produced at localized regions of mesenchyme near the tips of the lung buds creates a chemoattractant gradient that activates the FGFR2IIIb receptor in epithelial cells of the lung buds, inducing cell migration, differentiation, and proliferation required for branching morphogenesis. Deletion of Fgf10 or Fgfr2IIIb in mice blocked lung bud formation, resulting in pulmonary agenesis. Increased expression of FGF10 or FGF7 in the fetal mouse lung caused severe pulmonary lesions with all of the histologic features of cystic adenomatoid malformations. Actions of FGF10 in the mesenchyme are countered by expression of BMP-4 by epithelial cells in the lung buds that together control branching morphogenesis. Bidirectional paracrine signaling between mesenchymal and epithelial cells is mediated by a diversity of signaling networks. For example, FGF9, produced primarily by lung mesothelial and epithelial cells, is required for normal growth of the pulmonary mesenchyme. Examples of signaling polypeptides known to influence branching morphogenesis and differentiation of the respiratory tract are listed in Box 2.1 .
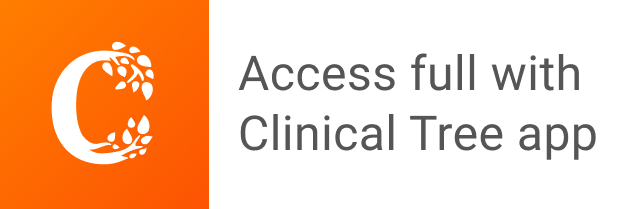