Chapter 6 Metabolism in Surgical Patients
Over recent decades, major progress has occurred in our understanding of the physiologic response to injury, increasing focus on improved nutrition in surgical care. Catabolism of skeletal muscle protein has been recognized as a major factor contributing to adverse outcomes following major surgery and trauma. In 1905, Sneve1 described the catabolic response as metabolic exhaustion and emaciation seen in burn patients. Cuthbertson2 studied the effects of long bone fractures in animal models, characterizing physiologic and metabolic responses into two phases: the early ebb phase and the flow phase. The former occurs the first several hours after injury, typically lasts 2 to 3 days, and is distinguished by reduced oxygen consumption (VO2), glucose tolerance, cardiac output, and basal metabolic rate. The latter typically starts several days after injury, lasts days to weeks, and features catabolic breakdown of skeletal muscle, negative nitrogen balance, hyperglycemia, and increased cardiac output, VO2, and respiratory rate.
In 1953 Cope and colleagues3 correlated muscle wasting following thermal injury with a measured rise in metabolic rate. Moore’s book, in 1959,4 proposed the use of continuous feeding to attenuate proteolysis and muscle catabolism observed following trauma. Establishing the feasibility of long-term parenteral nutrition (PN) in the late 1960s, Dudrick5 recognized that malnourished surgical patients with a preexisting protein deficit were at increased risk of complications.
It was then shown that the hypermetabolic response seen in patients with major burns resulted in part from an increase in catecholamine serum levels and that the metabolic response could be attenuated by ambient temperature and pharmacologic means. Further studies showed that severe trauma and burns lead to a hypercatabolic response, with deleterious effects on various organs, and that the inflammatory response contributes to hypermetabolism and subsequent catabolism seen following severe injury (Fig. 6-1).6 Modulation of this hypermetabolic response through pharmacologic and nutritional interventions, alteration of the environment, and improved surgical management are cornerstones of advancing surgical care.
Although widely quoted, the classic description of the ebb and flow response may not be apt for critically ill patients in a modern intensive care setting. A refined description has been proposed for these patients, who may suffer further insults from multiple operations or repeated bouts of sepsis (Fig. 6-2). The classic description may also oversimplify the array of responses that occur; these remain incompletely understood, particularly in the prolonged flow phase, which includes a short-term acute response and prolonged adaptive response.
Nutritional Requirements
The primary goal of nutritional support is to provide an adequate energy supply and all the nutrients necessary to support life and function. Nutrients in the diet require ingestion, digestion, absorption, and regulation before the substrates released are used, stored, or expended for energy. The major components of the diet are carbohydrates, lipids, and proteins (Fig. 6-3). As a source of calories, 1 g of carbohydrate yields 3.4 kcal (16 kJ) of protein, and 4 kcal (17 kJ) yields 9 kcal (37 kJ) of fat. Fuel preferences differ across various types of cells; erythrocytes and neurons use glucose preferentially, muscle and cardiac myocytes can also use fat, and enterocytes and lymphocytes can metabolize the amino acid glutamine. Adaptation to different fuels can occur under circumstances of starvation.
Carbohydrate Metabolism
In addition to glycogenolysis, glucose levels are maintained through the conversion of noncarbohydrate substrates by gluconeogenesis, which occurs primarily in the liver and, to a lesser extent, in the renal cortex. Substrates for this pathway include all amino acids except lysine and leucine, derived from the proteolysis of skeletal muscle, glycerol derived from the degradation of triglycerides (TGs) in adipose tissue, and lactate produced from anaerobic glycolysis (see Fig. 6-1). The enzyme-catalyzed reactions of the gluconeogenic pathway include the reversal of several steps of glycolysis and four irreversible reactions.
Lipid Metabolism
Lipolysis occurs within the cytosolic lipid droplets of adipocytes, in which a series of lipases initiate the breakdown of TAG into free fatty acids and glycerol. Hormone-sensitive lipase (HSL) was until recently thought to be the only enzyme to hydrolyze TGs in adipose tissue. A second enzyme, adipose triglyceride lipase (ATGL), is now believed to catalyze the first step in the hydrolysis of TGs (Fig. 6-4).7
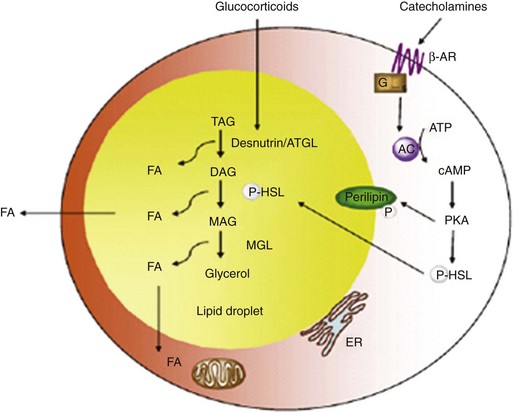
FIGURE 6-4 Regulation of lipolysis within adipocytes.
(Adapted from Ahmadian M, Wang Y, Sul HS: Lipolysis in adipocytes. Int J Biochem Cell Biol 42:555–559, 2010.)
Protein Metabolism
Human protein synthesis requires 20 amino acids; eight are termed essential amino acids because they cannot be synthesized de novo from other amino acids (10 if arginine and histidine are included as essential in infants) and must therefore be obtained in the diet. Six amino acids are termed conditionally essential amino acids because during childhood, illness, and other conditions, they may not be synthesized at rates that meet requirements and may therefore need to be supplemented. The remaining six are termed nonessential amino acids because they can be synthesized internally (Table 6-1).
AMINO ACID GROUP (ABBREVIATION) | FEATURES |
---|---|
Essential Amino Acids | Must be contained in diet because these cannot be synthesized |
Valine (Val) | Branched-chain amino acid |
Leucine (Leu) | Branched-chain amino acid |
Isoleucine (Ile) | Branched-chain amino acid |
Lysine (Lys) | |
Methionine (Met) | |
Threonine (Thr) | |
Phenylalanine (Phe) | |
Tryptophan (Trp) | |
Conditionally Essential | Conditionally indispensable because low synthesis rates may exceed requirements under certain conditions, especially in infants |
Arginine (Arg) | Essential depending on health status of individual and for infants because it cannot be synthesized quickly enough |
Histidine (His) | Previously considered essential for infants; now also considered essential for adults |
Tyrosine (Tyr) | Can be synthesized from phenylalanine |
Cysteine (Cys) | Can be synthesized from methionine |
Glutamine (Gln) | Major energy source for intestinal mucosa |
Proline (Pro) | |
Nonessential Amino Acids | Needs can be fully met by synthesis |
Alanine (Ala) | |
Asparagine (Asn) | |
Aspartate (Asp) | |
Glutamate (Glu) | |
Glycine (Gly) | |
Serine (Ser) |
Alanine is released from skeletal muscle, in addition to lactate, during the anaerobic glycolysis of glucose, which releases ATP. In the Cori cycle, the liver converts lactate produced by muscle back to glucose for muscle fuel in an ATP-dependent manner. Similarly, alanine can be used by the liver and is a preferred precursor for hepatic gluconeogenesis as part of the glucose-alanine cycle (see Fig. 6-1). Alanine is provided by either muscle during protein turnover or amino acids in the diet.
Metabolism of nitrogen-containing compounds in the body, including amino acids, produces ammonia, which is converted to urea, a less toxic substance, by a series of reactions that comprise the urea cycle. The urea cycle generates urea from ammonia produced from amino acid oxidation, in which certain amino acids enter the urea cycle directly as intermediates, including arginine. Increased catabolism of protein and the release of amino acids for gluconeogenesis lead to excess nitrogen production, negative nitrogen balance, and increased excretion of urea by the kidneys (see Fig. 6-1). Liver failure can lead to hepatic encephalopathy because of the buildup of nitrogenous compounds, including ammonia; inborn errors of metabolism also give rise to disorders from dysfunction of the urea cycle.
Regulation of the Amino Acid Pool
Glucose-Alanine and Glucose-Lactate Amino Acid Cycles
Lactate is a byproduct of the anaerobic metabolism of glucose. In physiologic states, it is produced by red blood cells (anaerobic cells) and skeletal muscle and taken up by the liver, where it is initially converted to pyruvate and subsequently to glucose via the gluconeogenic pathway. This is commonly known as the glucose-lactate, or Cori, cycle. The reactions that convert lactate back to glucose require a great deal of energy, which is supplied through lipolysis and β-oxidation of fat (see Fig. 6-1).
In the critically ill patient, lactate serves as a global marker of tissue hypoperfusion and insufficient oxygen delivery. However, there are additional mechanisms to explain lactate accumulation in these patients; elevations in plasma lactate levels in severely injured patients may in part be related to increases in glucose flux and may not totally be a reflection of a deficit in oxygen availability (Fig. 6-5).8
Intestinal Health
Following critical injury, there is thinning of the gut mucosa and a decrease in gut mucosal weight, protein, and DNA content, which are indicative of decreased cellular mass and absorptive surface of the small bowel, changes that result from an increased rate of small bowel mucosal apoptosis, with a relative decrease in small bowel cell proliferation.9 These changes have been associated with decreased absorption, deranged transportation, and increased gut permeability.
Protein Turnover
Protein turnover is continuously altered by dietary intake, synthesis, and protein breakdown. Amino acids are removed from the free pool of amino acids by protein synthesis and conversion to urea in a dynamic balance referred to as protein turnover. Net synthesis of protein is indicative of an anabolic state, whereas degradation of protein is indicative of a catabolic state. During critical illness, sepsis, trauma, or severe burn injury, there is an increased rate of synthesis and breakdown of muscle protein, although the magnitude of the latter is more significant and leads to a catabolic state.6 Increased proteolysis initiates an imbalance of the supply and demand of free amino acids; if protein breakdown persists, net protein catabolism leads to significant muscle wasting.
Proteolysis
Recent studies have proposed that Akt1 is the balancing force between muscle atrophy and hypertrophy. Insulin-like growth factor-1 (IGF-1) and other anabolic stimuli activate the PI3K-Akt1 pathway, leading to the activation of downstream targets (mTOR and S6K1) that stimulate muscle protein synthesis and hypertrophy (Fig. 6-6). Conversely, Akt1 is responsible for the phosphorylation status of the Foxo family of transcription factors. If Foxo is phosphorylated by Akt1, it leaves the nucleus and becomes inactive, thereby preventing the induction of atrophy. However, if Akt1 activity is suppressed, Foxo becomes dephosphorylated and transcriptionally active and directly binds the key atrogin-1 ubiquitination gene, among others, inducing increased protein degradation and muscle atrophy.
Nutritional Assessment and Monitoring
Nutritional assessment of surgical patients includes evaluation of preexisting malnutrition or obesity, medical conditions and metabolic disorders, malabsorption, dental disease, drug dependency, and alcoholism. In addition to requiring a comprehensive medical history and physical examination, nutritional assessment may include relevant laboratory tests, anthropometric measurements, and other assessments of body composition and energy expenditure, combined with the serial evaluation of results and response to therapy (Box 6-1). Malnutrition may exist primarily because of underlying pathology or inadequate intake, secondary to disease, trauma, and inflammatory processes or as a consequence of surgical interventions and operative procedures.
Box 6-1 Methods of Nutritional Assessment
Anthropomorphic measurements: IBW, BMI, skin fold thickness
Oxygen consumption, determination of respiratory quotient
Body composition analysis: Dual-energy X-ray absorptiometry
Biochemical measurements: albumin, transferrin, prealbumin
Measurement of nitrogen balance
Overfeeding is detrimental, leading to hypercapnia and metabolic acidosis, hyperglycemia, hypertriglyceridemia, hepatic dysfunction, and azotemia.10 Goal-directed nutritional support is essential for improving outcomes following trauma and surgery and should be based on repeated assessment of response to feeding. Nutritional support should be started as soon as possible if circumstances indicate that adequate oral intake will be unlikely for a patient within 5 days or if a preexisting nutritional deficit is present.
Malnutrition and Starvation
Up to 50% of patients admitted to the hospital may be malnourished11 and an additional 25% to 30% become malnourished during their hospital stay. Malnutrition can occur as a result of protein-calorie deficiency, predominant protein deficiency, and deficiency of specific micronutrients. Malnutrition can also result from a hypermetabolic state following trauma, critical illness, sepsis, severe burns, or major surgery. It leads to impairment of multiple organ systems, including the immune system, leading to an increased incidence of infection and delayed wound healing. Severe malnutrition and prolonged starvation eventually lead to reduced GI barrier function, respiratory insufficiency, skeletal muscle wasting, decreased myocardial mass, renal atrophy, diastolic cardiac dysfunction, and decreased sensitivity to inotropic agents.
Physical Body Measurements
Body Weight
Body weight reflects both fluid balance and nutritional status. Significant weight loss, particularly if rapid or unplanned, is a powerful predictor of mortality.12 Patients should be weighed daily, and accurate intake and output records should be maintained:
Anthropometric Measurements
Ideal Body Weight
A practical anthropomorphic approach is the calculation of IBW, particularly when the usual body weight or weight of the patient before the onset of illness is unknown. Values for IBW can be found in standardized tables that relate height to expected weight, or IBW can be estimated by the following equations13:
Evaluating Caloric Requirements
Energy Expenditure Equations
Several different equations are commonly used to estimate nutritional requirements. These formulas provide only an estimate because energy demands may vary considerably among patients and requirements will also depend on a patient’s condition and activity level. These formulas are based on parameters including age, gender, height, and weight. Examples include the Harris-Benedict,61 American College of Chest Physicians, Ireton-Jones (1997), Penn State (2003), and Swinamer (1990) equations. In severely burned patients, these and other equations can estimate nutritional requirements. Included in these other equations are the Curreri and Galveston formulas, which additionally take into consideration body surface area and burn percentage. It is important to select the appropriate equation based on age and correct estimate of degree of injury, because their inappropriate use can lead to significant overestimation of caloric needs and increased risk of overfeeding.
Harris-Benedict Equation
Most patients can be fed adequately by supplying 100% to 120% of the predicted REE as calculated by the Harris-Benedict equation.14 This equation estimates basal metabolic rate (BMR), assuming a normal resting physiologic state.
Therefore, multiplication by a stress factor is generally needed, usually ranging from 1.2 to 1.5 in ventilated patients with sepsis. A stress factor of 1.1 and 1.2 has been suggested for minor and major elective surgery, 1.35 and 1.6 for skeletal trauma and head injury, and 1.1, 1.5, and 1.8 for mild, moderate, and severe infection, respectively, has also been suggested.15 This may rise to 1.2 to 1.95 in burn injuries of 40% to 100% of total body surface area (TBSA).
Indirect Calorimetry
Measurements obtained are generally reliable and reproducible over a wide range of catabolic conditions, metabolic rates, and values of FIO2.Indirect calorimetry may also be used to monitor the adequacy of feeding by calculating the respiratory quotient (RQ = VCO2/ VO2) and evaluating substrate uptake. An RQ in the range of 0.7 to 1.0 is seen in the normal uptake of mixed substrates. An RQ of 0.7 or less is consistent with pure fat uptake and is indicative of underfeeding, whereas an RQ higher than 1.0 may indicate fat synthesis from carbohydrate and overfeeding. Overfeeding has been shown to be detrimental to critically ill patients and induces a rise in VCO2 because of increased lipogenesis.16 Such a rise in VCO2 may also contribute to difficult weaning from ventilatory support.
Poor agreement between measured and predicted REEs has been reported in certain circumstances, being as high as 635 ± 526 kcal/day in severely burned children.17 Bedside carts are therefore recommended to calculate optimal nutritional requirements in certain patient populations, including the following: (1) severely burned children; (2) ventilator-dependent patients; (3) patients with clinical signs of overfeeding or underfeeding; (4) patients with spinal cord injury or coma; (5) critically ill patients who are morbidly obese; and (6) those with failure to respond adequately to the use of diets determined according to equations, with failure determined by lack of improvement in clinical or biochemical nutritional measurements.
Monitoring Nutritional Status
Pediatric Assessment
Nutritional assessment in children, in addition to clinical history, physical examination, and biochemical markers, also includes plotting their growth on percentile charts. The Centers for Disease Control and Prevention (CDC) has published revised, standard, gender-specific percentile charts for growth, including stature and weight for age and BMI. These charts are demographically representative of the U.S. population from 2 to 20 years, with charts also available for younger children (Fig. 6-7). These charts are used to monitor a patient’s long-term nutritional progress. A patient’s position on the growth chart is the best simple tool to evaluate overall nutritional status in the acute setting. A value below the fifth percentile or a trend line crossing two major percentile lines indicates a serious failure to thrive.
Serum Proteins
A range of serum proteins are commonly used as indicators of nutritional status, with albumin being the most frequently used. Albumin accounts for more than 50% of the total protein in serum and is the major contributor to colloid osmotic pressure. An albumin level below 3 g/dL suggests suboptimal nutrition and has been used as the primary preoperative serum marker of malnutrition (Table 6-3). Albumin has a long half-life (t1/2), approximately 20 days, and perioperative albumin levels have been found to be a better prognostic indicator than anthropomorphic measurements for morbidity and mortality in surgical patients.18,19 Serum proteins with a shorter circulating duration are also used; these include transferrin (t1/2 = 10 days), prealbumin (t1/2 = 3 days), and retinol binding protein (t1/2 = 12 to 24 hours), because these are more sensitive indicators of recent changes.
Table 6-3 Surgical Risk by Serum Albumin Level
SERUM ALBUMIN (g/dL) | 30-DAY MORTALITY RATE (%) | 30-DAY MORBIDITY RATE (%) |
---|---|---|
>4.5 | ≤1 | ≤10 |
3.5 | 5 | 25 |
3.0 | 9 | 35 |
2.5 | 15 | 45 |
<2.1 | ≈30 | 65 |
Perioperative levels of serum albumin have been shown to be powerful predictors of morbidity and mortality.
Adapted from Gibbs J, Cull W, Henderson W, et al: Preoperative serum albumin level as a predictor of operative mortality and morbidity: Results from the National VA Surgical Risk Study. Arch Surg 134:36–42, 1999.
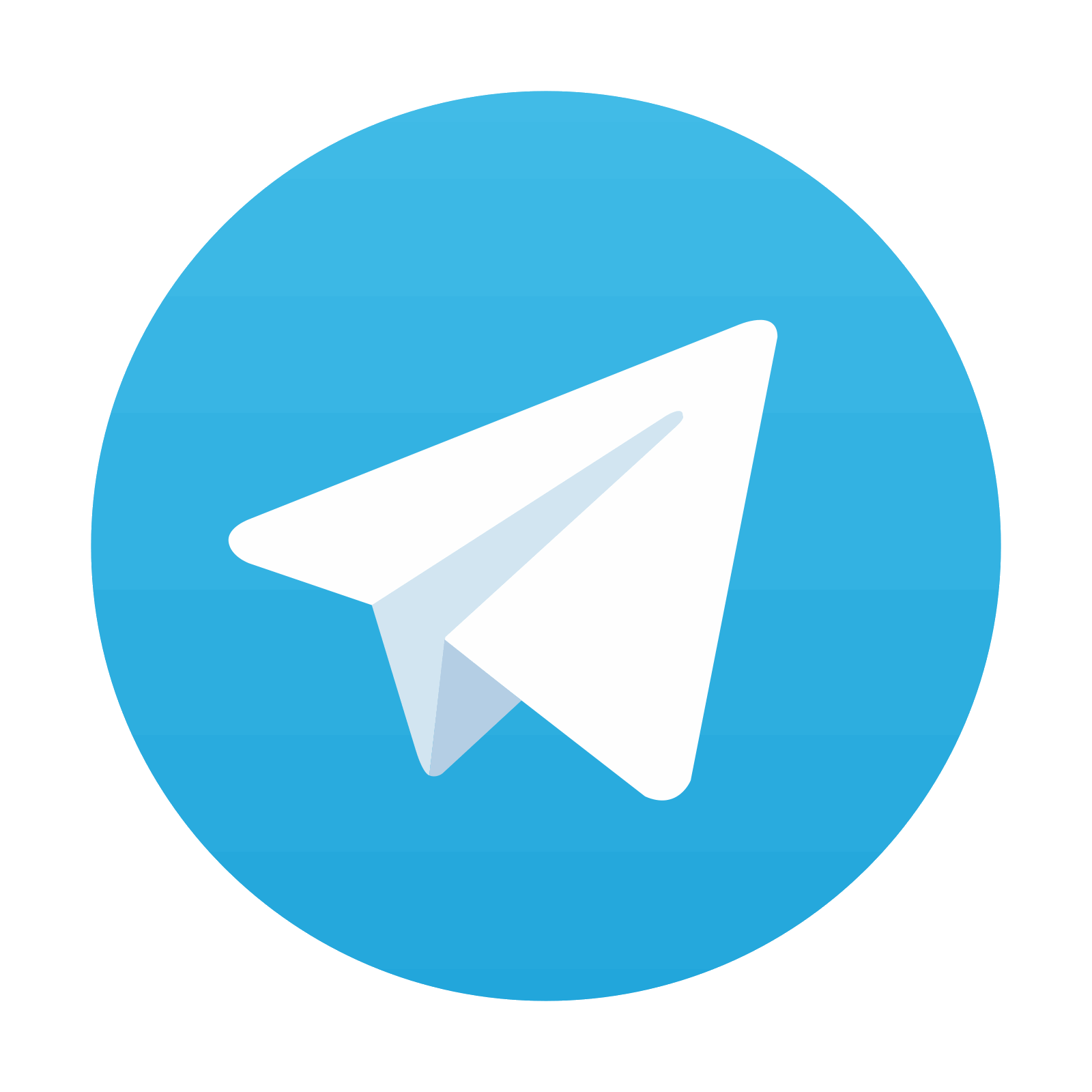
Stay updated, free articles. Join our Telegram channel
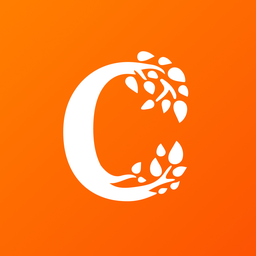
Full access? Get Clinical Tree
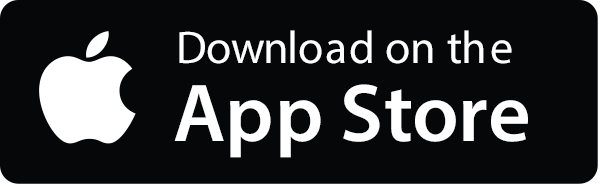
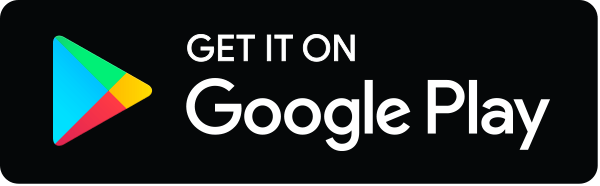