After reading this chapter you will be able to: Consensus exists among clinicians about the proper use of O2 therapy.1–4 As the primary member of the health care team responsible for O2 administration, the RT must be well versed in the goals and objectives of this therapy and its use in clinical practice. • Correct documented or suspected acute hypoxemia • Decrease symptoms associated with chronic hypoxemia • Decrease the workload hypoxemia imposes on the cardiopulmonary system In addition to relieving hypoxemia, O2 therapy can help relieve the symptoms associated with certain lung disorders. Specifically, patients with chronic obstructive pulmonary disease (COPD) and some forms of interstitial lung disease report less dyspnea when receiving supplemental O2.5 O2 therapy also may improve mental function among patients with chronic hypoxemia.6 Hypoxemia causes pulmonary vasoconstriction and pulmonary hypertension. Pulmonary vasoconstriction and hypertension increase workload on the right side of the heart. For patients with chronic hypoxemia, this increased workload over the long-term can lead to right ventricular failure (cor pulmonale). O2 therapy can reverse pulmonary vasoconstriction and decrease right ventricular workload.7 To guide practitioners in safe and effective patient care, the American Association for Respiratory Care (AARC) has developed and published clinical practice guidelines for O2 therapy. Excerpts from the AARC guideline on O2 therapy in acute care hospitals appear in Clinical Practice Guideline 38-1.2 Additional AARC guidelines for O2 therapy in the home or an extended care facility3 and for selection of O2 delivery devices for neonatal and pediatric patients4 are provided in Chapters 48 and 51. Laboratory measures for documenting hypoxemia include hemoglobin saturation and partial pressure of oxygen (PO2), as determined by either invasive or noninvasive means (see Chapter 18). Threshold criteria defining hypoxemia with these measures are described in the AARC clinical practice guideline (see Clinical Practice Guideline 38-1).2 O2 therapy is needed for patients with disorders associated with hypoxemia. Examples are postoperative patients; patients with carbon monoxide or cyanide poisoning, shock, trauma, or acute myocardial infarction, and some premature infants.1,2,8 Careful bedside physical assessment can disclose a patient’s need for O2 therapy. Table 38-1 summarizes the common respiratory, cardiovascular, and neurologic signs used in the detection of hypoxia. The RT combines this information with more quantitative measures such as arterial blood gas results to confirm inadequate oxygenation. The patient care team often relies on the RT to recommend administration of supplemental O2 based on quantitative and qualitative information. TABLE 38-1 Excerpts from the relevant AARC clinical practice guidelines (see Clinical Practice Guideline 38-1) outline the major precautions and hazards associated with administration of supplemental O2.2 Five of these hazards are common enough to warrant additional discussion. O2 toxicity primarily affects the lungs and the central nervous system (CNS).9–11 Two primary factors determine the harmful effects of O2: PO2 and exposure time (Figure 38-1). The higher the PO2 and the longer the exposure, the greater the likelihood of damage. Effects on the CNS, including tremors, twitching, and convulsions, tend to occur only when a patient is breathing O2 at pressures greater than 1 atm (hyperbaric pressure). Pulmonary effects can also occur with enriched O2 environments at normal atmospheric pressures. Table 38-2 summarizes the physiologic response to breathing 100% O2 at sea level. A patient exposed to a high PO2 for a prolonged period has signs similar to bronchopneumonia. Patchy infiltrates appear on chest radiographs and usually are most prominent in the lower lung fields. TABLE 38-2 Physiologic Responses of Healthy Individuals to Exposure to 100% Inspired Oxygen As the lung injury worsens, blood oxygenation deteriorates. If this progressive hypoxemia is managed with additional O2, the toxic effects worsen (Figure 38-2). However, if the patient can be kept alive while fractional inspired oxygen concentration (FiO2) is decreased, the pulmonary damage sometimes resolves. The toxicity of O2 is caused by overproduction of O2 free radicals. O2 free radicals are by-products of cellular metabolism. If unchecked, these radicals can severely damage or kill cells.9 Normally, however, special enzymes such as superoxide dismutase inactivate the O2 free radicals before they can do serious damage. Antioxidants such as vitamin E, vitamin C, and beta-carotene also can defend against O2 free radicals. Exactly how much O2 is safe is the subject of debate. Results of most studies indicate that adults can breathe up to 50% for extended periods without major lung damage.12 Rather than applying strict cutoffs, one can weigh both FiO2 and exposure time in assessing the risks of high PO2 (see the accompanying Rule of Thumb).13 The goal always should be to use the lowest possible FiO2 compatible with adequate tissue oxygenation. When breathing moderate to high O2 concentrations, a very small percentage of patients with COPD and chronic hypercapnia tend to ventilate less.14 Decreases in ventilation of nearly 20% have been observed in these patients with accompanying elevations in arterial partial pressure of carbon dioxide (PaCO2) of 20 to 23 mm Hg.15 However, this hypoventilation is not typical of patients with COPD, and although these patients should always be carefully monitored, appropriate management of hypoxemia should never be avoided because of concern for O2-induced hypoventilation. The primary reason some patients with COPD hypoventilate when given O2 is most likely suppression of the hypoxic drive. In these patients, the normal response to high partial pressure of carbon dioxide (PCO2) is blunted, the primary stimulus to breathe being lack of O2 as sensed by the peripheral chemoreceptors. The increase in the blood O2 level in these patients suppresses peripheral chemoreceptors, depresses ventilatory drive, and elevates the PCO2.16,17 High blood O2 levels may disrupt the normal ventilation/perfusion balance and cause an increase in dead space-to-tidal volume ratio (VD/VT) and in PaCO2.18 Retinopathy of prematurity (ROP), also called retrolental fibroplasia, is an abnormal eye condition that occurs in some premature or low-birth-weight infants who receive supplemental O2. An excessive blood O2 level causes retinal vasoconstriction, which leads to necrosis of the blood vessels. In response, new vessels form and increase in number. Hemorrhage of these delicate new vessels causes scarring behind the retina. Scarring often leads to retinal detachment and blindness.19 ROP most often affects neonates up to approximately 1 month of age, by which time the retinal arteries have sufficiently matured. Excessive O2 is not the only factor associated with ROP; other factors associated with ROP include hypercapnia, hypocapnia, intraventricular hemorrhage, infection, lactic acidosis, anemia, hypocalcemia, and hypothermia. Because premature infants often need supplemental O2, the risk of ROP poses a serious management problem. The American Academy of Pediatrics recommends keeping arterial PO2 in an infant less than 80 mm Hg as the best way to minimize the risk of ROP.8 FiO2 greater than 0.50 presents a significant risk of absorption atelectasis.20 Nitrogen normally is the most plentiful gas in both the alveoli and the blood. Breathing high levels of O2 quickly depletes body nitrogen levels. As blood nitrogen levels decrease, the total pressure of venous gases rapidly decreases. Under these conditions, gases that exist at atmospheric pressure within any body cavity rapidly diffuse into the venous blood. This principle is used for removing trapped air from body cavities. Giving patients high levels of O2 can help clear trapped air from the abdomen or thorax. This same phenomenon can cause lung collapse, especially if the alveolar region becomes obstructed (Figure 38-3). Under these conditions, O2 rapidly diffuses into the blood (see Figure 38-3, A). With no source for repletion, the total gas pressure in the alveolus progressively decreases until the alveolus collapses. Because collapsed alveoli are perfused but not ventilated, absorption atelectasis increases the physiologic shunt and worsens blood oxygenation.20 The risk of absorption atelectasis is greatest in patients breathing at low tidal volumes as a result of sedation, surgical pain, or CNS dysfunction. In these cases, poorly ventilated alveoli may become unstable when they lose O2 faster than it can be replaced. The result is a more gradual shrinking of the alveoli that may lead to complete collapse, even when the patient is not breathing supplemental O2 (see Figure 38-3, B). For an alert patient, this is not a great risk because the natural sigh mechanism periodically hyperinflates the lung. Despite numerous preventive measures, fires involving enriched O2 environments continue to occur in health care facilities. Fires seem to pose the greatest risk in operating rooms and in association with selected respiratory procedures. During surgery and procedures such as tracheotomies, electronic scalpels and similar devices are often used while the patient is receiving supplemental O2. To complicate matters, even higher O2 concentrations may exist under surgical drapes.21 Other situations associated with increased fire risk involve home care patients smoking while receiving low-flow O2 and the use of aluminum O2 regulators. Additionally, hyperbaric oxygen (HBO) therapy or therapy at increased atmospheric pressures (discussed later in this chapter) often involves the administration of supplemental O2 and greatly increases fire risk. Some simple strategies can be used to reduce the fire risk in health care facilities. Effectively managing the fire triangle of O2, heat, and fuel is key. An essential component is always using the lowest effective FiO2 for a given clinical situation. In addition, using scavenging systems to minimize O2 buildup beneath sterile drapes during surgery or while performing tracheostomies can help reduce fire risk. Avoiding the use of inappropriate or outdated equipment such as aluminum gas regulators and educating clinicians, patients and caregivers on safe O2 use are also important measures. Additionally, fire prevention protocols for HBO therapy should be strictly followed.21 RTs can select from an array of systems for administering O2 and other therapeutic gases. Proper device selection requires in-depth knowledge of both the general performance characteristics of these systems and the individual capabilities.22 O2 delivery devices traditionally are categorized by design. Three basic designs exist: low-flow systems, reservoir systems, and high-flow systems. Enclosures, commonly identified as a fourth category, are reservoirs that surround the head or body. The design categories share functional characteristics, capabilities, and limitations. Although design plays an important role in the selection of these devices, clinical performance ultimately determines how the device is used. The user judges the performance of an O2 delivery system by answering two key questions: (1) How much O2 can the system deliver (FiO2 or FiO2 range)? (2) Does the delivered FiO2 remain fixed or vary under changing patient demands?22 Whether a device delivers a fixed or variable FiO2 depends on how much of the patient’s inspired gas it supplies. If the system provides all of the patient’s inspired gas, FiO2 remains stable, even under changing demands. If the device provides only some of the inspired gas, the patient must draw the remainder from the surrounding air. In this case, the more the patient breathes, the more air dilutes the delivered O2, and FiO2 is lower. If the patient breathes less with this type of device, less air dilutes the O2, and FiO2 increases. A system that supplies only a portion of the inspired gas always provides a variable FiO2.23 FiO2 supplied with such systems can vary widely from minute to minute and even from breath to breath. Figure 38-4 shows these concepts as applied to low-flow, reservoir, and high-flow systems. With the low-flow system (see Figure 38-4, A) the patient’s inspiratory flow often exceeds the flow delivered by the device; the result is air dilution (shaded areas). The greater the patient’s inspiratory flow, the more air is breathed, and FiO2 is lower. The high-flow system (see Figure 38-4, B) always exceeds the patient’s flow and provides a fixed FiO2. A fixed FiO2 can be achieved with a reservoir system (see Figure 38-4, C), which stores a reserve volume (flow × time) that equals or exceeds the patient’s tidal volume. For a reservoir system to provide a fixed FiO2, the reservoir volume must always exceed the patient’s tidal volume, and there cannot be any air leaks in the system. Table 38-3 outlines the general specifications for the common O2 therapy systems in current use. TABLE 38-3 Overview of Oxygen Therapy Systems A nasal cannula is a disposable plastic device consisting of two tips or prongs approximately 1 cm long that are connected to several feet of small-bore O2 supply tubing (Figure 38-5). The user inserts the prongs directly into the vestibule of the nose while attaching the supply tubing either directly to a flowmeter or to a bubble humidifier. In most cases, a humidifier is used only when the input flow is greater than 4 L/min.2 Even with extra humidity, flow greater than 6 to 8 L/min can cause patient discomfort, including nasal dryness and bleeding.22 Cannulas should not be used in newborns and infants if their nasal passages are obstructed, and flows generally should be limited to 2 L/min unless a specialized high-flow cannula system is being used.2 A high-flow nasal cannula, which is a variation of a standard nasal cannula, is discussed in more detail later in this chapter. Table 38-3 lists the FiO2 range, FiO2 stability, advantages, disadvantages, and best use of a nasal cannula. Although use is generally limited to short-term O2 administration during specialized procedures such as a bronchoscopy, a nasal catheter is another low-flow O2 delivery device. A nasal catheter is a soft plastic tube with several small holes at the tip that is inserted by gently advancing it along the floor of either nasal passage and visualizing it just behind and above the uvula (Figure 38-6). Once in position, the catheter is taped to the bridge of the nose. If direct visualization is impossible, the catheter may be blindly inserted to a depth equal to the distance from the nose to the earlobe. When placed too deep, the catheter can provoke gagging or swallowing of gas, which increases the likelihood of aspiration. Nasal catheters also affect the production of secretions; for this reason, a nasal catheter should be replaced with a new one (placed in the opposite naris) at least every 8 hours. Nasal catheters should be avoided in most patients with maxillofacial trauma, basal skull fracture, nasal obstruction, and coagulation problems. It has also been determined that nasal catheters are inappropriate for neonatal patients. As a result of these notable limitations, nasal catheters are rarely used today.4 A transtracheal O2 catheter was first described by Heimlich in 1982.24 A physician surgically inserts this thin polytetrafluoroethylene (Teflon) catheter with a guidewire directly into the trachea between the second and third tracheal rings (Figure 38-7). A custom-sized chain necklace secures the catheter in position. Standard tubing connected directly to a flowmeter provides the O2 source flow.25 Because flow is so low, no humidifier is needed. Because the transtracheal catheter resides directly in the trachea, O2 builds up both there and in the upper airway during expiration. This process effectively expands the anatomic reservoir and increases the FiO2 at any given flow. Compared with a nasal cannula, a transtracheal catheter needs about half of the O2 flow to achieve a given arterial partial pressure of oxygen (PaO2).25 Some patients need a flow of only 0.25 L/min to achieve adequate oxygenation. This reduced flow can be of great economic and practical benefit to patients needing continuous long-term O2 therapy because it can greatly increase the duration of flow of portable O2 systems. Transtracheal O2 therapy can pose problems and risks, however, and these devices have not received widespread acceptance. Careful patient selection, rigorous patient education, and ongoing self-care with professional follow-up evaluation can help minimize these risks. Chapter 51 provides details on these aspects of transtracheal O2 therapy. Table 38-3 lists the FiO2 range, FiO2 stability, advantages, disadvantages, and best use of a transtracheal catheter. Research studies on nasal low-flow systems show O2 concentration ranging from 22% at 1 L/min to 60% at 15 L/min.2,3,22 The range of 22% to 45% cited in Table 38-3 is based on 8 L/min as the upper limit of comfortable flow. These wide FiO2 ranges occur because the O2 concentration delivered by a low-flow system varies with the amount of air dilution. The amount of air dilution depends on several patient and equipment variables. Table 38-4 summarizes these key variables and how they affect FiO2 provided by low-flow systems. TABLE 38-4 Variables Affecting FiO2 of Low-Flow Oxygen Systems Common problems with low-flow O2 delivery systems include inaccurate flow, system leaks and obstructions, device displacement, and skin irritation. The problem of inaccurate flow is greatest when low-flow flowmeters (≤3 L/min) are used. Given the trend toward assessment of outcome of O2 therapy (with either blood gases or pulse oximetry), ensuring the absolute accuracy of O2 input flow generally is not essential. Nonetheless, similar to all respiratory care equipment, flowmeters should be subjected to regular preventive maintenance and testing for accuracy. Equipment that fails preventive maintenance standards should be removed from service and repaired or replaced. Table 38-5 provides guidance on troubleshooting the most common clinical problems with nasal cannulas. Details on troubleshooting transtracheal catheters are provided in Chapter 51. TABLE 38-5 Troubleshooting Common Problems With a Nasal Oxygen Cannula Reservoir cannulas are designed to conserve O2 and are an alternative to the pulse-dose or demand-flow O2 systems described in Chapter 51. There are two types of reservoir cannula: nasal reservoir and pendant reservoir. Table 38-3 lists the FiO2 range, FiO2 stability, advantages, disadvantages, and best use of a reservoir cannula. A nasal reservoir cannula operates by storing approximately 20 ml of O2 in a small membrane reservoir during exhalation (Figure 38-8). The patient draws on this stored O2 during early inspiration. The amount of O2 available increases with each breath and decreases the flow needed for a given FiO2. Although the device is comfortable to wear, many patients object to its appearance and may not always comply with prescribed therapy. The pendant reservoir system helps overcome esthetic concerns by hiding the reservoir under the patient’s clothing on the anterior chest wall (Figure 38-9). Although the device is less visible, the extra weight of the pendant can cause ear and facial discomfort. At low flow, reservoir cannulas can reduce O2 use 50% to 75%. A patient at rest who needs 2 L/min through a standard cannula to achieve an arterial oxygen saturation (SaO2) greater than 90% may need only 0.5 L/min through a reservoir cannula to achieve the same blood oxygenation. During exercise, reservoir cannulas can reduce flow needs approximately 66%; the savings is approximately 50% at high flow.26 Although flow savings is predictable, factors such as nasal anatomy and breathing pattern can affect the performance of the device. For these devices to function properly at low flow, patients must exhale through the nose (this reopens or resets the reservoir membrane). In addition, exhalation through pursed lips may impair performance, especially during exercise. For these reasons, prescribed flow settings should be individually determined by means of clinical assessment, including SaO2 monitoring, during rest and exercise.26 The low flow at which the reservoir cannula operates makes humidification unnecessary. Excess moisture can hinder proper action of the reservoir membrane.26 Even regular use can cause membrane wear. For this reason, patients should replace the reservoir cannula approximately every 3 weeks. Replacement needs partially offset the O2 cost savings afforded by these devices. Masks are the most commonly used reservoir systems. There are three types of reservoir masks: (1) simple mask, (2) partial rebreathing mask, and (3) nonrebreathing mask. Table 38-3 lists the FiO2 range, FiO2 stability, advantages, disadvantages, and best use of each of these devices. A simple mask is a disposable plastic unit designed to cover both the mouth and the nose (Figure 38-10). The body of the mask itself gathers and stores O2 between patient breaths. The patient exhales directly through open holes or ports in the mask body. If O2 input flow ceases, the patient can draw in air through these holes and around the mask edge. The input flow range for an adult simple mask is 5 to 10 L/min. Generally, if flow greater than 10 L/min is needed for satisfactory oxygenation, use of a device capable of a higher FiO2 should be considered. At a flow less than 5 L/min, the mask volume acts as dead space and causes carbon dioxide (CO2) rebreathing.27 Because air dilution easily occurs during inspiration through its ports and around its body, a simple mask provides a variable FiO2. How much FiO2 varies depends on the O2 input flow, the mask volume, the extent of air leakage, and the patient’s breathing pattern.28 As shown in Figure 38-11, a partial rebreathing mask and a nonrebreathing mask have a similar design. Each has a 1-L flexible reservoir bag attached to the O2 inlet. Because the bag increases the reservoir volume, both masks provide higher FiO2 capabilities than a simple mask. The key difference between these designs is the use of valves. A partial rebreathing mask has no valves (see Figure 38-11, A). During inspiration, source O2 flows into the mask and passes directly to the patient. During exhalation, source O2 enters the bag. However, because no valves separate the mask and the bag, some of the patient’s exhaled gas also enters the bag (approximately the first third). Because it comes from the anatomic dead space, the early portion of exhaled gas contains mostly O2 and little CO2. As the bag fills with both O2 and dead space gas, the last two-thirds of exhalation (high in CO2
Medical Gas Therapy
Describe when oxygen (O2) therapy is needed.
Assess the need for O2 therapy.
Describe what precautions and complications are associated with O2 therapy.
Select an O2 delivery system appropriate for the respiratory care plan.
Describe how to administer O2 to adults, children, and infants.
Describe how to check for proper function and to identify and correct malfunctions of O2 delivery systems.
Describe how to evaluate and monitor a patient’s response to O2 therapy.
Describe how to modify or recommend modification of O2 therapy on the basis of patient response.
Describe how to implement protocol-based O2 therapy.
Identify what indications, complications, and hazards apply to hyperbaric O2 therapy.
Identify when and how to provide nitric oxide therapy.
Oxygen Therapy
General Goals and Clinical Objectives
Decreasing Symptoms of Hypoxemia
Minimizing Cardiopulmonary Workload
Clinical Practice Guideline
Assessing the Need for Oxygen Therapy
Finding
Mild to Moderate
Severe
Respiratory
Tachypnea
Tachypnea
Dyspnea
Dyspnea
Paleness
Cyanosis
Cardiovascular
Tachycardia
Tachycardia, eventual bradycardia, arrhythmia
Mild hypertension, peripheral vasoconstriction
Hypertension and eventual hypotension
Neurologic
Restlessness
Somnolence
Disorientation
Confusion
Headaches
Distressed appearance
Lassitude
Blurred vision
Tunnel vision
Loss of coordination
Impaired judgment
Slow reaction time
Manic-depressive activity
Coma
Precautions and Hazards of Supplemental Oxygen
Oxygen Toxicity
Exposure Time (hr)
Physiologic Response
0-12
Normal pulmonary function
Tracheobronchitis
Substernal chest pain
12-24
Decreasing vital capacity
25-30
Decreasing lung compliance
Increasing P(A-a)O2
Decreasing exercise PO2
30-72
Decreasing diffusing capacity
Depression of Ventilation
Retinopathy of Prematurity
Absorption Atelectasis
Fire Hazard
Oxygen Delivery Systems: Design and Performance
Category
Device
Flow
FiO2 Range
FiO2 Stability
Advantages
Disadvantages
Best Use
Low flow
Nasal cannula
-8 L/min (adults) ≤2 L/min (infants)
22%-40%
Variable
Use on adults, children, infants; easy to use; disposable; low cost; well tolerated
Unstable, easily dislodged; high flow uncomfortable; can cause dryness, bleeding; polyps; deviated septum and mouth breathing may reduce FiO2
Patient in stable condition who needs low FiO2; home care patient who needs long-term therapy, low to moderate FiO2 while eating
Nasal catheter
-8 L/min
22%-45%
Variable
Use on adults, children, infants; good stability; disposable; low cost
Difficult to insert; high flow increases back pressure; needs regular changing; polyps, deviated septum may block insertion; may provoke gagging, air swallowing, aspiration
Procedures in which cannula is difficult to use (bronchoscopy); long-term care of infants
Transtracheal catheter
-4 L/min
22%-35%
Variable
Lower O2 use and cost; eliminates nasal and skin irritation; improved compliance; increased exercise tolerance; increased mobility; enhanced image
High cost; surgical complications; infection; mucous plugging; lost tract
Home care or ambulatory patients who need increased mobility or do not accept nasal O2
Reservoir cannula
-4 L/min
22%-35%
Variable
Lower O2 use and cost; increased mobility; less discomfort because of lower flow
Unattractive, cumbersome; poor compliance; must be regularly replaced; breathing pattern affects performance
Home care or ambulatory patients who need increased mobility
Simple mask
5-10 L/min
35%-50%
Variable
Use on adults, children, infants; quick, easy to apply; disposable; inexpensive
Uncomfortable; must be removed for eating; prevents radiant heat loss; blocks vomitus in unconscious patients
Emergencies; short-term therapy requiring moderate FiO2; mouth breathing patients requiring moderate FiO2
Partial rebreathing mask
Minimum of 10 L/min (prevent bag collapse on inspiration)
40%-70%
Variable
Same as simple mask; moderate to high FiO2
Same as simple mask; potential suffocation hazard
Emergencies; short-term therapy requiring moderate to high FiO2
Nonrebreathing mask
Minimum of 10 L/min (prevent bag collapse on inspiration)
60%-80%
Variable
Same as simple mask; high FiO2
Same as simple mask; potential suffocation hazard
Emergencies; short-term therapy requiring high FiO2
Nonrebreathing circuit (closed)
3 × VE (prevent bag collapse on inspiration)
21%-100%
Fixed
Full range of FiO2
Potential suffocation hazard; requires 50 psi air/O2; blender failure common
Patients who need precise FiO2 at any level (21%-100%)
High flow
AEM
Varies; should provide output flow >60 L/min
24%-50%
Fixed
Easy to apply; disposable, inexpensive; stable, precise FiO2
Limited to adult use; uncomfortable, noisy; must be removed for eating; FiO2 >0.40 not ensured; FiO2 varies with back pressure
Patients in unstable condition who need precise low FiO2
Air-entrainment nebulizer
10-15 L/min input; should provide output flow of at least 60 L/min
28%-100%
Fixed
Provides temperature control and extra humidification
FiO2 < 0.28 or >0.40 not ensured; FiO2 varies with back pressure; high infection risk
Patients with artificial airways who need low to moderate FiO2
Blending system (open)
Should provide output flow of at least 60 L/min
21%-100%
Fixed
Full range of FiO2
Requires 50 psi air/O2; blender failure or inaccuracy common
Patients with high Ve who need high FiO2
High-flow nasal cannula system
Up to 40 L/min (depending on system)
35%-90%
Variable or fixed depending on system and input flow
Wide range of FiO2 and relative/absolute humidity; use on adults, children, infants
FiO2 not ensured depending on input flow and patient breathing pattern; infection risk
Patients of all ages with high or variable Ve who need supplemental O2, positive pressure, or humidity
Enclosure
Oxyhood
≥7 L/min
21%-100%
Fixed
Full range of FiO2
Difficult to clean, disinfect
Infants who need supplemental O2
Isolette
8-15 L/min
40%-50%
Variable
Provides temperature control
Expensive, cumbersome, unstable FiO2 (leaks); difficult to clean, disinfect; limits patient mobility; fire hazard
Infants who need supplemental O2 and precise thermal regulation
Tent
12-15 L/min
40%-50%
Variable
Provides concurrent aerosol therapy
Expensive, cumbersome, unstable FiO2 (leaks); requires cooling; difficult to clean, disinfect; limits patient mobility; fire hazard
Toddlers or small children who need low to moderate FiO2 and aerosol
Low-Flow Systems
Nasal Cannula
Nasal Catheter
Transtracheal Catheter
Performance Characteristics of Low-Flow Systems
Increases FiO2
Decreases FiO2
Higher O2 input
Lower O2 input
Mouth-closed breathing*
Mouth-open breathing*
Low inspiratory flow
High inspiratory flow
Low tidal volume
High tidal volume
Slow rate of breathing
Fast rate of breathing
Small minute ventilation
Large minute ventilation
Long inspiratory time
Short inspiratory time
High I : E ratio
Low I : E ratio
Troubleshooting Low-Flow Systems
Problem or Clue
Cause
Solution
No gas flow can be felt coming from the cannula
Flowmeter not on
System leak
Adjust flowmeter
Check connections
Humidifier pop-off is sounding
Obstruction distal to humidifier
Find and correct the obstruction
Flow is set too high
Use alternative device
Obstructed naris
Use alternative device
Patient reports soreness over lip or ears
Irritation or inflammation caused by appliance straps
Loosen straps
Place cotton balls at pressure points
Use a different device
Mouth breathing
Habitual mouth breathing, blocked nasal passages
Switch to simple mask or Venturi mask
Reservoir Systems
Reservoir Cannula
Reservoir Masks
Stay updated, free articles. Join our Telegram channel
Full access? Get Clinical Tree
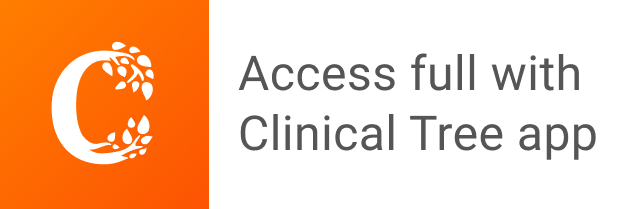