Magnetic resonance imaging (MRI) in clinical use relies on two fundamental principles: the excess of hydrogen molecules in water contained in the tissues of the human body (a) and (b) the phenomenon of magnetic resonance, specifically the magnetlike behavior of these protons that causes them to align and spin at a predictable frequency within an external magnetic field. This alignment occurs when a stationary field is applied by the superconducting magnet as the patient lies within the bore. For each imaging sequence, a smaller secondary set of coils within the bore produce rapid bursts of gradient magnetic fields that cause protons to transiently tilt off-axis. As these protons relax from their high-energy state to their original alignment within the static magnetic field, a radiofrequency signal is emitted from the patient and absorbed by the receiving coils strapped directly to the patient in the anatomic area being imaged (Figure 18-1). The characteristics of this signal are deciphered by the computer using complex algorithms to generate an image.
Although MRI has an excellent signal-to-noise ratio (SNR) allowing for superb tissue differentiation, additional clinical information is commonly obtained by using intravenous contrast agents. While several classes have been developed, only gadolinium-based agents are in clinical use in peripheral arterial imaging. Gadolinium is a toxic element, requiring a chelating agent before it can be employed in medical use. Once injected intravenously, it specifically shortens the T1 relaxation period of protons, resulting in an increased signal seen as a brightened structure on the MR image. Consequently, it has become an indispensable tool for imaging the vascular system. Compared with iodinated x-ray contrast, gadolinium-based agents are relatively benign and adverse reactions are rare. A relatively new manifestation of gadolinium-associated adverse reactions, nephrogenic systemic fibrosis (NSF), has been observed in patients with renal disease who receive contrast for MRI studies. Patients primarily experience thickening and tightening of the skin, but reports also describe involvement of the liver, lungs, muscles, and heart. Thus far, only 57 cases have been reported to the Food and Drug Administration, but these have prompted recommendations to minimize the use of gadolinium contrast agents in patients with advanced kidney disease as much as possible.1,2
While the lack of ionizing radiation in MRI compares favorably to x-ray methods, it does carry its own specific safety concerns. The large, superconducting magnet has potential to attract and/or interfere with all ferromagnetic objects within a sufficient distance. These include equipment within the MRI suite (oxygen canisters, IV poles, etc.) and devices implanted within the patient (pacemakers, defibrillators, TENS units, etc). It has been established that most types of cardiovascular stents, prosthetic heart valves, orthopedic implants, and surgical clips are safe, although in all instances, physician discretion is required to insure patient safety. While recent data have demonstrated that patients with pacemakers and defibrillators can be scanned safely with specific monitoring safeguards, these devices remain a strong relative contraindication to MRI.
The application of MRI to the peripheral arterial system has evolved significantly because of improvements in hardware and scanning techniques. In particular, high-performance magnetic field gradients allow very rapid image acquisition, and contrast-enhanced (CE) imaging techniques have dramatically increased spatial resolution and the amount of data that can be acquired. Magnetic resonance angiography (MRA), in particular, offers several advantages when compared to conventional x-ray angiography or computed tomography angiography (CTA). MRA is minimally or noninvasive, acquires three-dimensional (3D) information, allows evaluation of structures outside of the vessel lumen, and perhaps most importantly, requires no radiation exposure or nephrotoxic, iodinated contrast.
There are several techniques used to perform an MR examination of the peripheral arterial system. These include time-of-flight (TOF), phase contrast (PC), black-blood, and contrast-enhanced MRA (CE-MRA) techniques. While these techniques are vastly different, all can be used to create MR images of the vasculature and use gradient-recalled echo (GRE) sequences. More recently, single-shot balanced, steady-state free precession techniques (SSFP) have been employed as a rapid means of evaluating the vasculature.
Time-of-Flight MRA. Of the three MRA techniques, TOF has been in clinical use the longest and is still frequently used to image the intracranial vasculature.3,4,5 TOF imaging requires both suppression of background signal and generation of signal from flowing blood. Rapid slice-selective radiofrequency excitation pulses are used to saturate the signal from stationary tissue, making them appear dark. Blood flowing into the imaging slice appears bright because it has not been exposed to the saturation pulse. The signal intensity of blood in TOF imaging increases as the flow velocity in the vessel increases. Problems can arise in TOF imaging when blood flow is so slow that blood exposed to the saturation pulse does not exit the imaging volume. TOF can also be difficult when imaging tortuous vessels or vessels that are in the same plane as the imaging volume because of signal from the remaining suppressed blood.
Because arteries and veins usually travel together, signal from veins can obscure visualization of the adjacent arteries. To suppress the signal in the veins, a saturation pulse is applied above the desired imaging volume such that blood in veins will travel into the imaging volume without signal.3,4 Similar techniques can be used to suppress unwanted signal because of motion of the diaphragm during respiration or the heart during the cardiac cycle.
TOF can be performed as either a two-dimensional (2D) or 3D acquisition. Where 3D acquisition is best performed in areas with relatively high flowing blood, 2D acquisitions are best in areas of slow flowing blood or areas that could be affected by respiratory motion. 3D TOF is higher resolution than 2D TOF and is less susceptible to signal loss in areas of turbulent flow.
Phase-Contrast MR. Phase contrast MR (PC-MR) uses the differences in speed, or phase shift, between magnetic spins moving in blood and spins in stationary tissue.6,7 In contrast to TOF and CE-MRA techniques, PC-MR can be used to quantify flow velocities in addition to being used to generate angiographic images. Quantification of flow velocities with PC-MRA can be performed because the phase shift between mobile and stationary magnetic spins is linearly dependent on the flow velocity.
PC-MR is predominantly used to identify direction of flow and to quantify flow velocities and volumes through a specified area (Figure 18-2). It also has the advantage, compared to TOF, of improved background suppression, allowing imaging of smaller vessels and larger volumes, in addition to improved imaging of vascular structures with slow flow, such as vascular malformations and veins. However, PC-MR sequences usually have longer acquisition times and are more sensitive to magnetic field heterogeneities.
FIGURE 18-2.
MIP image (A) from a contrast-enhanced MRA performed in a patient with a history of repaired coarctation of the aorta. Magnitude (B) and phase contrast (C) images obtained at the level of the coarctation repair (line on image A). By placing regions of interest over the vessel on the images throughout the cardiac cycle and measuring the velocity at each phase, one can generate velocity-time or flow-time curves (D).

Dark-Blood Imaging. Dark-blood MR imaging techniques are used to image the vessel wall because of the high-contrast resolution of these sequences (Figure 18-3). Presaturation bands are used to null the signal of flowing blood within a vessel. As a result, high-velocity flowing blood will appear black because of the lack of signal as the blood flows into the imaging volume. Because of this, dark-blood techniques can suffer from artifacts when blood flow is slow, such as in a large aneurysm, when a patient has a very low cardiac output, or in the false lumen of a dissection. In these cases, slow flowing blood may mimic thrombosis or other pathologic findings.
Contrast-Enhanced MRA. With the development of CE-MRA techniques, MR angiography has significantly improved in quality and clinical acceptance.8,9 CE-MRA sequences exploit the effect of gadolinium-based contrast agents on the T1 relaxation time of blood, greatly increasing the intensity of the detected signal compared to surrounding structures. Successful CE-MRA requires synchronization of image acquisition to the first pass of contrast material through the vessels of interest. As a result, extremely fast imaging sequences are used to acquire a 3D volume data set.
Several strategies have been implemented for timing of image acquisition. Test bolus injection of a small volume (2–3 mL) of contrast allows calculation of the delay from the time of injection to the time of contrast arrival in the region of interest. Another technique uses rapid, thin-slice acquisition to automatically detect the arrival of contrast prior to initiation of 3D CE-MRA acquisition.10,11 More recently, dynamic, time-resolved CE-MRA techniques have been developed that allow one to obtain multiple 3D volumes, similar to conventional angiography12,13,14 (Figure 18-4). After acquiring the images, one can select the 3D volume that best demonstrates the vessel of interest. This last technique is useful when flow is asymmetric or to assess vessels at different points in time.15
While CE-MRA techniques are less susceptible to artifacts than other MRA sequences, artifacts do occur.16,17 As with all MR sequences, CE-MRA is prone to susceptibility artifacts from metallic devices i.e., clips, stents, and prostheses. The degree of artifact depends on the ferromagnetic content of the material used to make the device. Incorrect timing of image acquisition can lead to opacification of veins in addition to arteries. If too many veins are opacified, evaluation of the arteries can be more difficult. Wraparound artifact occurs when the imaged volume does not include the entire volume of the patient’s body at that level. If not enough contrast material is used, the SNR may be insufficient to accurately evaluate vascular anatomy.
Steady-State Free Precession. SSFP is a noncontrast, bright-blood cine imaging technique utilized to view tissue morphology and blood flow through vascular structures. It is most commonly employed as an adjunct to dark-blood, PC-MR, and CE-MRA techniques and is an improvement from earlier forms of cine imaging using gradient-recalled echo sequences. Areas of high-velocity flow may produce some artifact, but it is a relatively simple and reproducible technique yielding quality motion images.
Because multiple image sets are usually acquired during an MRA study, several methods have been developed to present the data in a manner more familiar to the clinician. However, any finding detected on these manipulated images needs to be confirmed on the original source images. Methods used to reconstruct the images include multiplanar reformatted (MPR) (Figure 18-5), maximum intensity projection (MIP), and volume rendered (VR) methods18 (Figure 18-6). MPR involves reconstruction of the 3D data set on a computer workstation in any imaging plane, permitting the viewer to display the vessel along its longitudinal axis. Because vessels often curve in and out of a given plane, MIP methods combine data from multiple planes to produce images resembling those of conventional angiography. A limitation of MIP images is related to the fact that low-signal-intensity pixels at the edge of a vessel are lost because of automatic thresholds used in the image reconstruction. Therefore, the lumen diameter will appear smaller than in actuality, contributing to the overestimation of the degree of stenosis often noted in MRA. VR, or surface rendered, reconstructions are most useful for viewing the surface of vessels and for evaluating the 3D relationships of vessels and their surrounding structures.
TOF, PC, and CE-MRA techniques are used to evaluate the intracranial and cervical vasculature. TOF-MRA can be performed as a 2D or 3D acquisition. 2D TOF-MRA involves the acquisition of multiple thin, contiguous, or overlapping slices, which are then combined to create a volume of MRA data. The images can then be viewed in an axial plane or by using image projection techniques mentioned previously, such as MIP, MPR, or VR. While 2D TOF is very sensitive to slow flowing blood, it can be very time consuming to perform. 3D TOF-MRA is faster than 2D TOF and has higher spatial resolution and more optimal SNR. However, 3D TOF is less sensitive to slow flowing blood. Another TOF technique, known as multiple overlapping thin-slab TOF combines aspects of both 2D and 3D methods. Multiple thick slabs are acquired contiguously and combined to create the full volume.
PC-MRA can produce cross-sectional images superior to those produced with TOF imaging because of the superior background suppression with PC imaging. In addition, PC-MRA allows one to calculate flow velocities through stenoses. However, these sequences are more susceptible to patient motion and artifacts related to turbulent flow than TOF sequences and take longer to acquire than comparable TOF-MRA sequences.
CE-MRA is predominantly used to evaluate the aortic arch and cervical vessels.19 Bolus-timing or time-resolved CE-MRA techniques can be used for cervical and intracranial CE-MRA. Newer, stronger magnetic field gradients permit more rapid MRA acquisition, permitting imaging of exclusively arterial phase studies.
MRA is frequently performed at the same time as MRI of the brain in patients who have had a stroke or transient ischemic attack (TIA) to evaluate for the presence of occlusive atherosclerotic disease. A combination of TOF and CE-MRA techniques are used to visualize the intracranial vasculature20 (Figures 18-7 and 18-8). MRA has a reported sensitivity of 80% to 100% and specificity of 80% to 99% for detecting stenoses of 50% or greater in the proximal intracranial circulation. As in other areas of the body, MRA of the intracranial arteries tends to overestimate the degree of stenosis. In areas of focally severe stenosis, TOF-MRA can make a vessel appear occluded because of spin dephasing and acceleration in the area of stenosis.
FIGURE 18-8.
(A) Contrast-enhanced MRA of the cervical and intracranial circulation in a patient with an occluded right internal carotid artery, just distal to the carotid artery bifurcation (arrow). The dashed line represents the expected location of the occluded right internal carotid artery. (B) The right middle cerebral artery (open arrow) fills via flow from the right posterior communicating artery (arrowheads).

Although MRA is an attractive modality for identifying intracranial aneurysms because it is relatively noninvasive and does not use ionizing radiation,21,22 it is somewhat limited by slightly lower-spatial resolution than conventional angiography and CTA. The spatial resolution of 3D TOF-MRA techniques is approximately 0.8 mm. It is commonly used to image the circle of Willis and to detect small (3–5 mm) intracranial aneurysms. The ability of MRA to detect aneurysms smaller than 3 mm is still limited, although studies have shown that these rarely rupture. MRA is also limited in its ability to accurately detect and define small branch vessels that can arise from or near intracranial aneurysms. However, evaluation of giant saccular aneurysms is an area where MRA may be superior to digital subtraction angiography (DSA).
Despite the previously mentioned limitations, MRI of the head and MRA of the head and neck are crucial in the preintervention evaluation of patients with intracranial aneurysms (Figure 18-9). MRA has also been used to screen patients with an increased risk of intracranial aneurysms: patients with polycystic kidney disease, cerebral arteriovenous malformations (AVM), fibromuscular dysplasia, aortic coarctation, connective tissue disorders, and a family history of subarachnoid hemorrhage or aneurysms. Previous retrospective and prospective studies evaluating the sensitivity and specificity of MRI and MRA for the detection of intracranial aneurysms have found sensitivities of 69% to 99% and a specificity of 100%.22 Sensitivies are lower for smaller aneurysms. Newer, faster CE-MRA techniques can image the intracranial circulation with contrast opacification of the arteries without venous contamination. Also, MRA at 3.0 T may be more sensitive at detecting small aneurysms than MRA at 1.5 T.23,24
MRI and MRA are routinely used to follow patients who have had surgical or percutaneous interventions for intracranial aneurysms.25,26 Because the clips and endovascular coils used to treat aneurysms are not ferromagnetic, MR imaging is not affected by artifact as is seen with CT scan angiography in these patients.
3D TOF and CE-MRA techniques can be used to diagnose and characterize intracranial vascular malformations. In the case of AVM, MRA is used to identify feeding arteries, AVM nidus, and draining veins and to accurately measure the size of the lesion. MRI most accurately depicts the relationship of the AVM to the adjacent cerebral anatomy and the best 3D representation of the malformation. Limitations of MRA in evaluating AVMs include imaging flow in tortuous feeding arteries, differentiating blood flow from methemoglobin in a subacute hematoma, and poor conspicuity of slower flowing venous blood distal to the AVM. Despite these limitations, MRA is the preferred imaging modality for the pretreatment evaluation of patients with AVMs.
MRA of the carotid arteries was initially performed using 2D and 3D TOF sequences. These techniques have been largely replaced with CE-MRA techniques, which have short image acquisition times and are less prone to overestimation of stenosis. Both timing-bolus and time-resolved techniques of performing CE-MRA have been evaluated for the detection and grading of carotid artery stenosis. These techniques are attractive because of their ability to image the carotid arteries without venous contamination. The sensitivity and specificity of MRA for carotid artery atherosclerotic disease are above 90%.27,28,29,30 In addition to atherosclerotic disease, CE-MRA plays a significant role in the diagnosis of extracranial arterial dissections, allowing accurate visualization of intimal flaps and luminal flow31 (Figure 18-10).
FIGURE 18-10.
Axial source images from a 3D time-of-flight MRA (A-C) in a patient with a right internal carotid artery (ICA) dissection demonstrate asymmetry in the caliber of right and left ICAs, right smaller than left (arrows); crescent-shaped hypointense signal surrounding the true lumen of the right ICA (A, B, asterisks) corresponding to slow flow in the false lumen; and a linear signal void within the right ICA (C, arrowhead), corresponding to the intimal flap. Contrast-enhanced MRA in the same patient reveal irregularity in the caliber of the right ICA (D) as a result of the dissection (open arrow).
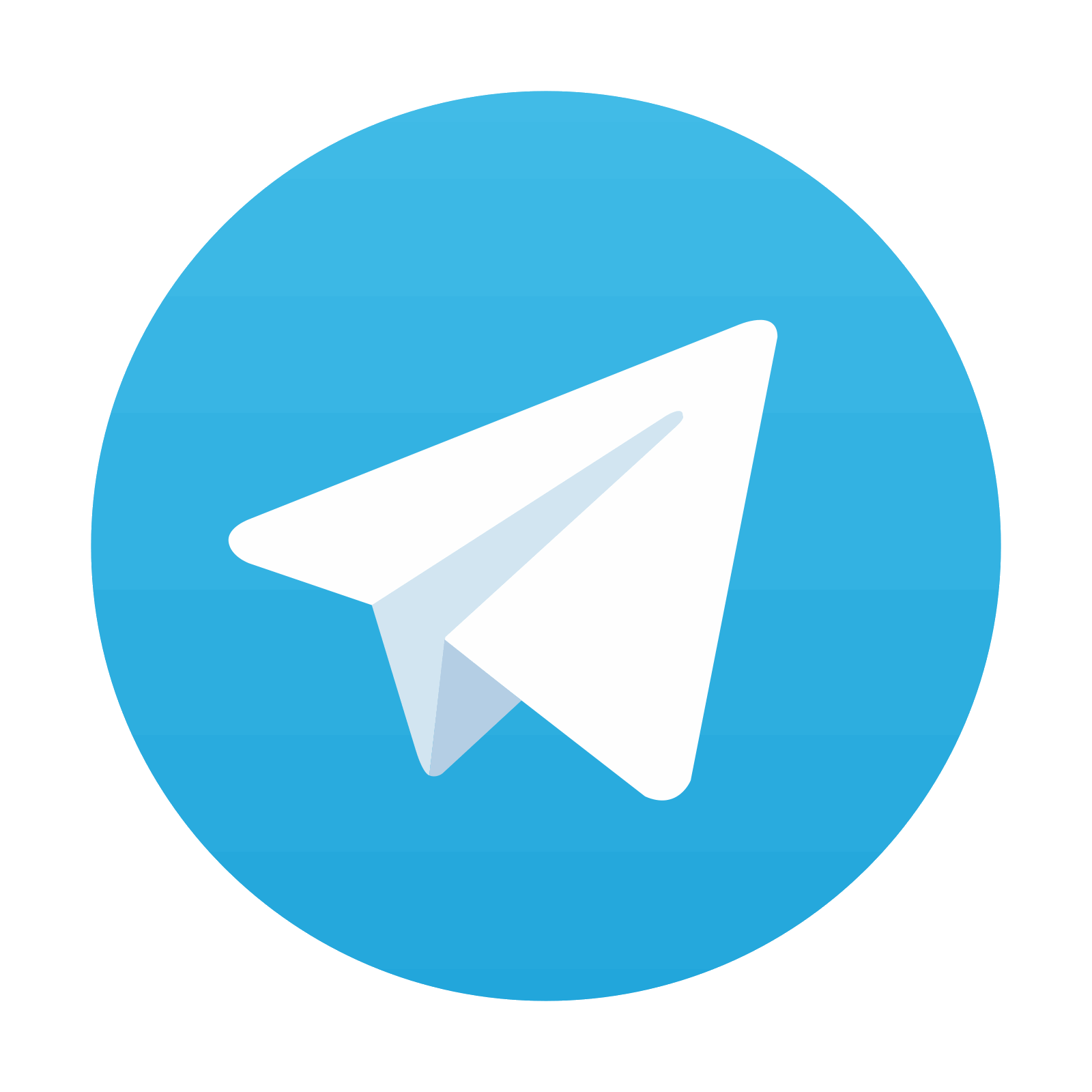
Stay updated, free articles. Join our Telegram channel
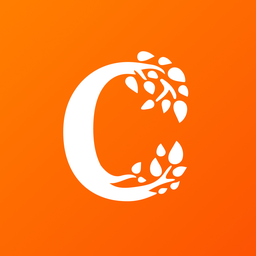
Full access? Get Clinical Tree
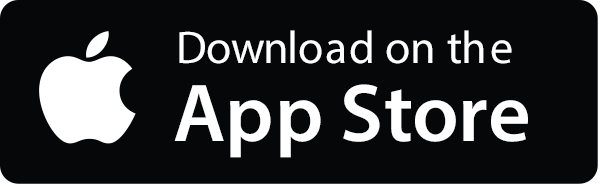
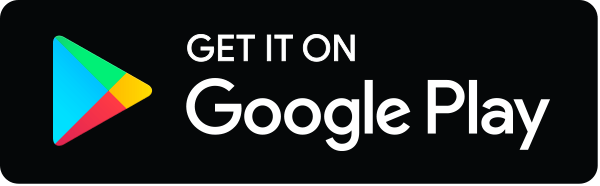
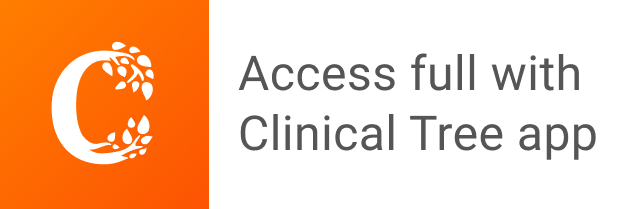