div epub:type=”chapter” role=”doc-chapter”>
5. Extravascular Lung Water Monitoring
5.1 Definition and a Brief History
Water contributes 80% of the lung components; however, most of them existed in the blood vessels and distributed in a gradient of increasing density from nondependent to dependent regions. By definition, extravascular lung water (EVLW) is the amount of water that is contained in the lungs outside the pulmonary vasculature, composed of the sum of interstitial, intracellular, alveolar, and lymphatic fluid; however, pleural effusions did not include [1]. The EVLW monitoring and evaluation play an essential role in pulmonary edema assessment.
5.2 Physiology of Lung Water

The basic principle of the Starling’s law, EVLW is the result of balance between the hydrostatic and oncotic pressure across the capillary and alveolus, and is influenced by drainage of the lymph
The doctrine status of the Starling model has been challenged for a long time. Danielli found there was an endothelial surface layer lining the luminal side of the capillary endothelium in 1940 [3]. Recent studies found a nonlinear relationship between hydrostatic pressure and vascular permeability [4, 5]. Endothelial glycocalyx (EG), which composed of glycosaminoglycans and proteins and coating the surface of endothelial-like a gel, is believed to play a crucial role in extravasation prevention [6]. Acting as a molecular sieve, EG can limit water and solute efflux across the intercellular junction. Furthermore, EG provides scaffolding on which serum plasma proteins accumulate and a layer of ultrafiltrate formed, which pulls fluid to the intravascular compartment with the powerful oncotic force. Additionally, EG transmitting the shear stress from blood flow to the endothelium cytoskeleton and initiating intracellular signaling as a mechanosensor, increasing capillary permeability [7].
The understanding of the pathophysiology of pulmonary edema goes further based on the emerging role of EG. It is well acknowledged now that excessive fluid extravasation could be induced by either a significant increase of capillary hydrostatic pressure or the damage of the EG layer, which is frequently seen in trauma and ischemia/reperfusion injuries.
The pulmonary lymphatics serve as a drainage system, which presents along the peribronchovascular, interlobular septa, and the pleural spaces are responsible for EVLW clearance by actively removing fluid and solutes from the interstitial tissue and poured into the superior vena cava through the thoracic duct continuously. Zarins demonstrated that the lymph flow is about 20 mL/h normally and could increase 5–10 times to compensate for the increase of interstitial pressure [8]. Gee reported that when transpulmonary pressure was increased to 20 cmH2O, water content contained by the peribronchovascular cuffs increased by 70% [9]. Further extravasation of interstitial fluid is limited due to the progressive decrease of the interstitial compartment compliance. This protection could be destroyed due to the breakdown of interstitial proteoglycans, which caused loss of matrix integrity.
The dynamic equilibrium between the fluid leakage and lymphatic drainage is essential to maintain the dryness of the lungs, and hence preserve the normal function of oxygenation.
In terms of the movement of extravasated fluid into the alveoli from the interstitium, the process is quite faster. The mechanisms of alveolar fluid clearance (AFC) is responsible for the removal of excess fluid from the alveoli to the interstitial tissue across the alveolar epithelial barrier, in which the active ion transport plays a key role. The sodium permeant ion channels expressed in the distal lung epithelium are responsible for actively transport sodium back into the interstitial space, with chlorine and water following. Other molecular transporters including but not limiting the cystic fibrosis transmembrane conductance regulator, Na+-K+-ATPase, and several aquaporin water channels. The process of isosmolar fluid transport across the distal lung epithelium is upregulated by both catecholamine-dependent and -independent mechanisms [10].
The AFC rate depends on the species difference. In human beings, roughly on the order of 25% per hour [11], whereas in mice may be as high as 50% per hour [12].
Regulated with the mechanisms described above, EVLW seldom exceeds 500 ml in normal lungs; however, it may be 75–100% higher in the condition of alveolar flooding. Typically, increased interstitial EVLW is caused by increased hydrostatic pressure in the pulmonary capillaries (as occurs in congestive heart failure), decreased oncotic pressure (such as hypoalbuminemia), or the increased permeability of the alveolocapillary barrier (as in the ARDS) [13]. The accumulation of EVLW in interstitial spaces will thicken the blood-air barrier (0.5–2 μm in the normal range), impede the gas exchange under the same alveolar-arterial difference of oxygen, and reduce lung compliance.
It is well known that one of the major pathophysiological characteristics of ARDS is the increase of pulmonary permeability and the increase of hydrostatic pressure also prevalent due to fluid resuscitation. In the early phase of ARDS, the increase in interstitial EVLW could be compensated by the enhancement of lymphatic drainage. Once the speed of drainage is overwhelmed by high filtration rate and fluid enters the alveoli, the impaired active ion transport and lymphatic drainage network may not clear excess fluid effectively, leading to the accumulation of EVLW, and what is worse is the flood of alveoli. Apart from ARDS, an increase of EVLW is also seen in septic shock [14] and other critically ill patients.
Indexed to the body weight (mL/kg), instead of absolute value (ml), EVLWI is better correlated with the oxygenation and the lung injury score, provide more accurate information in predicting the mortality of patients with ARDS. After screening a series of autopsies in Japan from more than 800 hospitals between 2004 and 2009, Tagami and colleagues compared the postmortem lung weights, and converted to EVLW with the following equation [15]:
![$$ \mathrm{EVLW}\ \left(\mathrm{mL}\right)=\left[0.56\times \mathrm{lung}\ \mathrm{weight}\ \left(\mathrm{g}\right)\right]-58.0. $$](https://i0.wp.com/thoracickey.com/wp-content/uploads/2021/07/480284_1_En_5_Chapter_TeX_Equa.png?w=960)
Their results showed the mean value of EVLWI was 7.3 ± 2.8 mL/kg in 534 normal lungs, and 13.7 ± 4.5 mL/kg in 1688 lungs with diffuse alveolar damage (DAD), respectively. The cut-off value higher than 10 mL/kg could establish the diagnosis of DAD with a sensitivity of 81.3% and a specificity of 81.2%, therefore lower than 10 mL/kg is regarded as the normal values of EVLWI.
It will be a great value to detect and quantify the EVLW in monitoring the progression of the illness, evaluating the severity, and accessing treatment response accurately and timely. The histologic and gravimetric methods are good for experimental study; however, they could not be used in the clinical scenario. Ideally, a technique that fulfills the advantage of noninvasive, easy to implement, reproducible, and less expensive concomitantly is preferred by clinicians, which may provide enough information with sufficient sensitivity and specificity.
5.3 The Methods of EVLW Measuring
5.3.1 Gravimetry
Gravimetry remains as the golden standard in EVLW measuring. It is a precisely laboratory postmortem technique, which measures the total lung water by weighting the difference of lungs before and after desiccation. The amount of intravascular lung water could be estimated by comparing the hematocrit in systemic blood and lung specimen, assuming red blood cells do not cross the alveolar-capillary barrier, although it is not always true. Subtracting intravascular lung water from total lung water, we could know the EVLW.
5.3.2 Imaging Methods
The information provided by all imaging methods is spatially related, which means that each pixel (picture) or voxel (volume) in a cross-sectional image of the lung corresponding to a specific physical volume. Different from other solid organs, the lung is air-containing and the parenchyma in a specific region varies with the state of lung inflation. The signal must be integrated over the entire lung to quantify changes in images of EVLW.
It has to be pointed out that imaging methods (except for positron emission tomography) just provide a rough estimation of total water content or concentration, and could neither differentiate extravascular water from vascular water technically, nor extracellular from intracellular water. If the blood volume varies with the hemodynamic status, the data calculated from imaging methods may mislead the estimation of EVLW.
5.3.3 Chest Radiography
Conventional chest roentgenogram is fast and easy to acquire method for detecting the presence of pulmonary edema, describing the overall distribution with the lung, and semi-quantifying the amount of exudate fluid. The typical signs such as pulmonary “congestion,” vascular “redistribution,” peribronchial cuffing, perihilar “haze,” septal (Kerley) lines, and “interstitial” pattern to the radiographic densities may indicate the modest increases in EVLW (more than 35%) [16]. Further increase of EVLW presents with acinar opacities, ground-glass opacities, and frank consolidations. Initially, the distribution of increased radiographic density may be prominent regionally, i.e., gravity-dependent lung regions, and even turned to be opacity with the progression of fluid exudation.
In the clinical environment, the degree of interobserver variability and the lack of sensitivity raised concerns about the accuracy of chest radiography monitoring. For example, the interobserver variability ranged from 36% to 71% in diagnosing ARDS based on the American-European Consensus Conference definition, after reviewing 28 chest radiographs by 21 radiology experts [17]. Compared with CT, the accuracy of chest radiography was only 72% in alveolar-interstitial pulmonary edema assessment [18].
Apart from the limitations mentioned above, sometimes the X-rays are difficult to interpret, which is often the case in supine ICU patients, influenced by the heart and relaxed diaphragm. Besides, repeated radiation exposure brings safety risks to chest X-ray photography, all of which require the use of more sensitive and accurate techniques when evaluating EVLW.
5.3.4 Computed Tomography
Computed tomography (CT) enables the visualization of lung lesions from the apex to the bottom, from the anterior to the posterior regions, and quantify the infiltrate density of the lungs. In transverse sections of CT display, the arbitrary Hounsfield units (HU) calibrated against substances of known density, could easily define the spatial distribution of edema. Normally the value of 0 HU characterizes a voxel with a density equal to that of water and the value of −1000 HU characterizes a voxel with a density equal to that of air. In isolated canine lungs, CT densitometry can detect modest increases in EVLW [19].
The disadvantages of CT include exposure to large doses of ionizing radiation (i.e., pregnant women or preterm infants), time-consuming, unrepeatable, and facing the risk of critically ill patient’s transportation. Different from the previous studies, the most recent study questioned the diagnostic value of quantitative CT analysis for the assessment of pulmonary fluid status in unselected critically ill patients, mainly contribute to the real clinical routine other than a standardized protocol, i.e., without an end-expiratory pause while receiving mechanical ventilation [20].
5.3.5 Lung Ultrasonography
Although echocardiography is widely used in the ICU, the lung was not considered suitable for this imaging technology for a long time. Clinicians started the exploration of lung ultrasound since 1989 and gradually turned it to be a valuable point-of-care (POC) tool in the assessment of acute pulmonary diseases [21]. Healthy lung tissue is poorly penetrated by ultrasound due to the high acoustic impedance of air, which is usually defined as “black” lung. However, in the condition of increased EVLW, the air-fluid interface between collapsed, fluid-filled, and aerated alveoli will result in the acoustic reverberation artifacts, which provide the possibility of transmission of ultrasound deep into the diseased lung tissues.

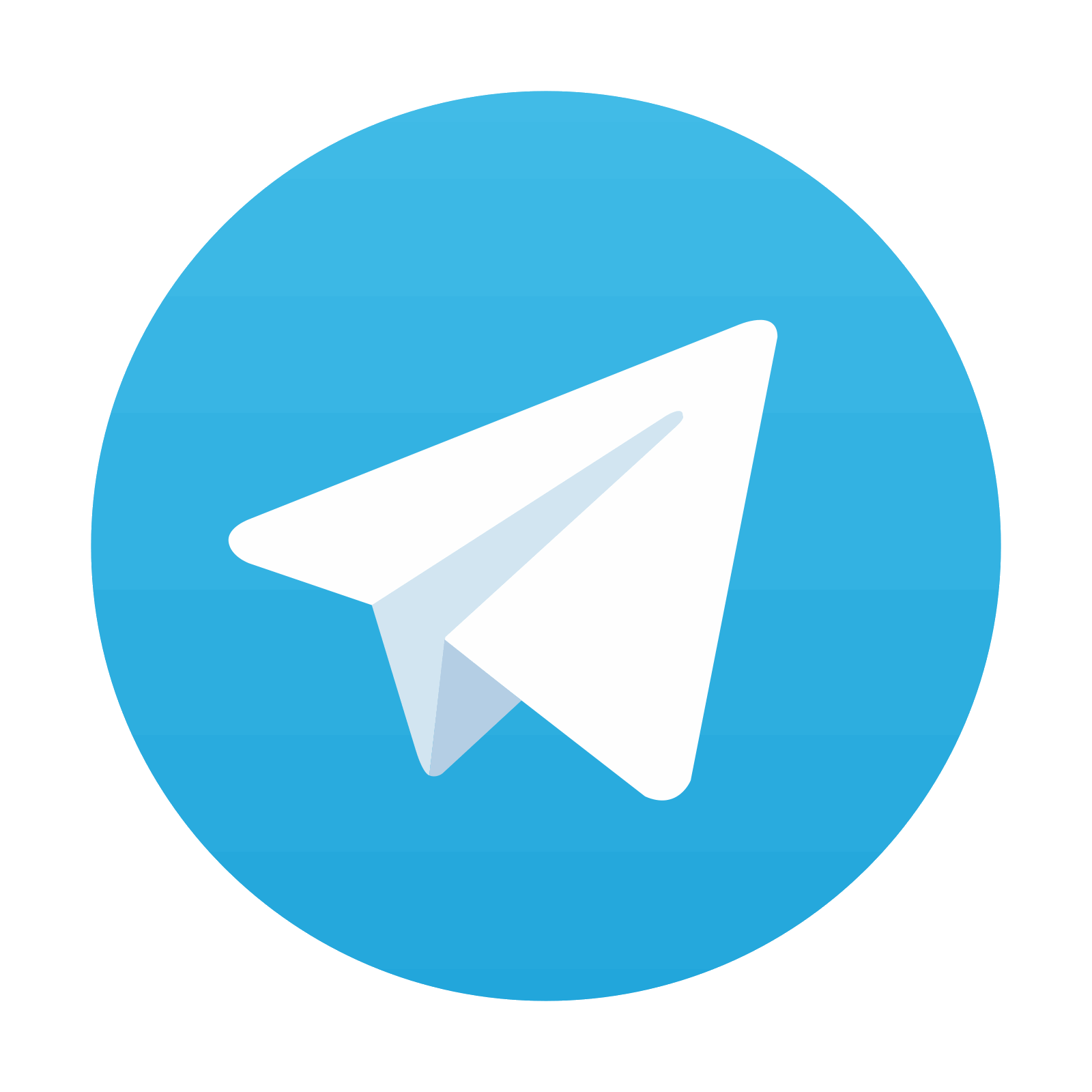
Stay updated, free articles. Join our Telegram channel
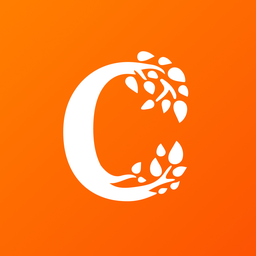
Full access? Get Clinical Tree
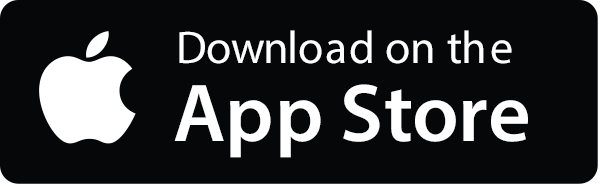
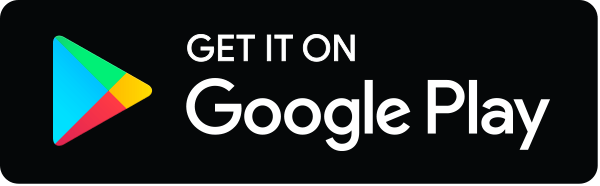