Fig. 19.1
Groups of nerve fibers are bundled together , along with capillaries and fibroblasts, to create a fascicle. Each fascicle is further surrounded by the perineurium, a dense connective tissue layer. Fascicles are then bundled together and encased by another layer of dense connective tissue called the epineurium
Peripheral nerves are classified by their conduction velocity, size, and function. Increased conduction velocity is associated with both increased nerve fiber diameter and myelin. Myelin improves nerve electrical insulation and expedites rapid impulse propagation through saltatory conduction. Similarly, myelinated nerve fibers commonly have a diameter exceeding 1 micron. Motor and sensory functions requiring critical speed are usually associated with large-diameter, myelinated fibers know as A-fibers [2]. A-alpha and A-beta fibers are the largest with rapid conduction velocities (2–33 micron diameter, 30–120 m/sec). These fibers function for motor and proprioception providing both afferent and efferent innervation for muscles and joints. Slightly smaller A-gamma fibers are still fairly rapid (3–6 microns, 15–35 m/sec) and provide muscle tone through efferent innervation of the muscle spindle. Similarly, A-delta fibers (1–4 microns, 5–25 m/sec) provide afferent innervation to sensory nerves for pain, touch, and temperature. Autonomic functions are more commonly relayed by small-diameter fibers. The smallest myelinated fibers, B-fibers (<3 microns, 3–15 m/sec), provide preganglionic sympathetic innervation. C-fibers are the smallest fibers (0.3–1.3 microns) and the only unmyelinated fibers. They provide postganglionic sympathetic and afferent sensory nerve innervation for autonomic functions, pain, and temperature.
With advanced age, changes occur to both the CNS and PNS. The spinal vertebral bodies are brittle and may stimulate bone overgrowth. This results in vertebral disc space loss. These changes may lead to increased pressure and compression on both spinal cord and spinal nerve roots as they exit to the periphery. Peripherally, while some atrophy occurs with time, peripheral nerve impulse conduction is primarily slowed due to myelin degradation. Myelin degradation is attributed to multiple factors including bone overgrowth causing nerve compression and decreased blood flow to the nerve. Additionally, chronic medical conditions , such as diabetes, may exacerbate nerve injury. While peripheral nerve axons have some capacity to repair themselves in younger patients when the proximal nerve cell body is unharmed, this repair process is decreased and often incomplete in the geriatric population. CNS changes may create balance instability, decreased strength, and radiculopathy, while PNS changes are associated with delayed reflexes and decreased sensation [3].
Basic Neuronal Physiology
Stimulation of sensory nerves by a thermal, chemical, or mechanical stimulus will trigger receptors at the distal ends of sensory nerves. Sufficient stimulus will create an electrical current known as an action potential. Action potentials are momentary, localized episodes of depolarization in which a positive charge is conducted along nerves by the movement of sodium ions across the nerve membrane down both electrical and chemical gradients. This creates a reversal in the electrical polarity of the membrane generating electrical current.
At rest, ion channels establish electrical and chemical gradients across the nerve membrane (Fig. 19.2). Active Na+/K+ ATPase channels pump sodium ions out of the cell and potassium ions into the cell, with a 3:2 ratio, respectively [4]. This creates a chemical gradient with a high intracellular potassium concentration and a high extracellular sodium concentration. Concurrently, passive ion channels allow free movement of ions across membranes, facilitating extracellular movement of potassium along a concentration gradient. In addition to this chemical gradient, the active pumping of positive ions (sodium) out of the cell combined with the passive extracellular leakage of positive ions (potassium) creates an electrical gradient. This results in a resting electrical potential difference with the inside of the cell having a negative charge (−70 to −90 mV) compared to the outside cell.


Fig. 19.2
At rest, electrical and chemical gradients across the nerve membrane are established by ion channels. Active Na+/K+ ATPase channels pump sodium ions out of the cell and potassium ions into the cell with a 3:2 ratio, respectively. Passive ion channels allow potassium movement out of the cell (concentration gradient). Active pumping of sodium combined with passive leakage of potassium ions out of the cell creates an electrical gradient, resulting in a resting electrical potential difference with the inside of the cell having a negative charge (−70 to −90 mV)
In addition to passive and active ion channels, voltage-gated sodium channels located on the nerve membrane open and close in response to the membrane potential difference. These voltage-gated sodium channels consist of an α-subunit and one or two β-subunits [5]. Nerve membrane stimulation triggers the α-subunit to go through multiple conformational changes, including four functional states (resting, activated, inactivated, and deactivated). Simplistically, the channel can be considered to have two functional gates , an inner gate (h) and outer gate (m). When the nerve membrane is at resting potential (−70 to −90 mV), the outer m-gate is open and the inner h-gate is closed. With activation, the outer m-gate opens creating a rapid influx of sodium ions (electrical and chemical gradients) and the membrane potential increases. If sufficient sodium channels open to raise the membrane potential greater than −60 mV, a widespread opening of sodium channels is triggered resulting in an even more rapid influx of sodium ions. If the membrane potential bypasses neutral to reach +20 mV, the inner h-gates close and the sodium channels become inactivated, preventing further ion movement [6]. The membrane depolarization creates a potential difference, relative to adjacent areas, generating an electrical current and elevating the membrane potentials of contiguous areas. This triggers a wave of depolarization of the adjacent nerve membranes in unmyelinated nerves and adjoining nodes of Ranvier in myelinated nerves.
After the membrane depolarization peaks (+50 mV), sodium influx stops, potassium efflux ensues, and repolarization reverses the membrane potential. The nerve is refractory to further stimulation during the inactivated and deactivated states , preventing rapid depolarization of the axonal section and inhibiting retrograde impulse conduction. During the inactivated phase, sodium ions do not move through the voltage-gated channels. However, they are shifted to the extracellular space by the Na+/K+ ATPase pump. Movement of potassium through the passive ion channels further helps restore the membrane potential. As the membrane reaches −60 mV, the outer m-gate opens and the voltage-gated sodium channel is reactivated.
Pharmacology
Mechanism of Action
Local anesthetics are most commonly thought to block nerve conduction by reversibly binding with one or more α-subunits on voltage-gated sodium channels at an intracellular location (Fig. 19.3) [7]. Local anesthetics are typically manufactured as water-soluble salts (usually hydrochlorides) in an acidic solution. They must be converted into a non-ionized, lipid-soluble form in order to diffuse across a lipophilic lipoprotein membrane and enter the cell. The proportion of local anesthetic transformed to the non-ionized form is correlated with both tissue pH and drug ionization constant (pKa). After the local anesthetic moves into the intracellular space, a decreased intracellular pH regenerates the ionized form, which binds to the α-subunit and blocks the sodium channel. If enough sodium channels are interrupted, threshold potentials are not achieved and impulse conduction is obstructed. Intracellularly, ionized local anesthetic may also further disrupt the intra-membrane portion of the sodium channel, and this may be augmented by blockade of potassium channels, calcium channels and G-protein-coupled receptors [8–10]. Other theorized mechanisms of local anesthetic action exist. Local anesthetics may alter conduction by disrupting surface membrane charge. The Meyer-Overton theory proposes that local anesthetics result in cell membrane expansion which then impedes sodium conductance.


Fig. 19.3
Injected local anesthetics exist in an ionized, water-soluble (LA-H+) quaternary form. In order to transverse the lipid bilayer, it must change to the non-ionized, lipophilic (LA) tertiary form. The drug then changes back into the ionized form (LA-H+) in order to bind to the voltage-gated sodium (Na+) channels
Local anesthetic affinity varies with the state of the sodium channel. Affinity is highest when the sodium channel is opening (activated or inactive). Affinity is least when the channel is closed (deactivated or resting). Consequently, a resting nerve is less sensitive to local anesthetic than a nerve that is frequently stimulated.
As small nerve fibers are more vulnerable to blockade compared to large fibers, neural blockade is first noticed for the sensation of pain and temperature followed by touch, deep pressure, and last motor. Interrupting conduction is faster in smaller fibers due to shorter axonal length. Large fibers (touch, pressure, and motor) require higher concentrations to produce adequate blockade compared to small myelinated fibers (pain). However, local anesthetics block myelinated fibers more rapidly than unmyelinated fibers since drug pools near the axonal membrane. Consequently, C-fibers, which are small and unmyelinated, are difficult to block. Unfortunately, these afferent postganglionic fibers of the autonomic nervous system carry information about pain, touch, and warmth and are associated with neuropathic pain when damaged.
Lastly, local anesthetics differ in their affinity for the receptor. Lidocaine binds and dissociates rapidly, while bupivacaine dissociates slower. This is related to chemical differences between various local anesthetics.
Local Anesthetic Types and Metabolism
Local anesthetics are composed of a lipophilic aromatic ring connected to a terminal amine by either an ester or amide linkage (Fig. 19.4). Local anesthetics are classified as either esters or amides based on this intermediate chain (Table 19.1).


Fig. 19.4
Local anesthetics are composed of a lipophilic aromatic ring connected to a terminal amine by either an ester or amide linkage. Local anesthetics are classified as either esters or amides based on the intermediate chain
Table 19.1
Local anesthetics: classifications and characteristics
Medication | pKa | Onset (minutes) | Lipid solubilitya | Partition coefficientb | Potency | Protein binding | Duration (hours) |
---|---|---|---|---|---|---|---|
Amides | |||||||
Lidocaine | 7.7 | 10–20 Fast | 2.9 | 43 | Intermediate | 64 | 2–5 Intermediate |
Mepivacaine | 7.8 | 10–20 Fast | 0.8 | 21 | Intermediate | 77 | 2–5 Intermediate |
Prilocaine | 7.9 | 10–20 Fast | 25 | Intermediate | 55 | 2–5 Intermediate | |
Etidocaine | 7.7 | 10–20 Fast | 141 | 800 | Intermediate | 94 | |
Articaine | 7.8 | 10–20 Fast | – | Intermediate | 95 | 2–5 Intermediate | |
Bupivacaine | 8.2 | 15–30 Intermediate | 28 | 346 | High | 95 | 5–15 Long |
Levobupivacaine | 8.1 | 15–30 Intermediate | 346 | High | 96 | 5–15 Long | |
Ropivacaine | 8.0 | 15–30 Intermediate | 3 | 115 | High | 94 | 5–15 Long |
Esters | |||||||
Procaine | 9 | Slow | 1.7 | Low | 6 | 0.5–1.5 Short | |
Chloroprocaine | 9.3 | 10–15 Fast | 0.14 | 810 | Intermediate | – | 0.5–1.5 Short |
Cocaine | 8.7 | Slow | – | High | 98 | Long | |
Benzocaine | 3.5 | Slow | – | – | – | – | |
Tetracaine | 8.6 | Slow | 4.1 | 221 | Intermediate | 76 | Long |
The intermediate chain also determines the mechanism of metabolism and elimination. Esters are hydrolyzed in plasma by pseudocholinesterase, and pseudocholinesterase deficiencies will prolong neural blockade. The type of substitution and location on the aromatic ring determines the rate of hydrolysis. Consequently, procaine is hydrolyzed four times faster than tetracaine. Conversely, amides are metabolized by the liver in a dealkalization reaction. Hepatic function and blood flow determine amide clearance, and decreases in these will increase the elimination half-life.
Decreases in lean tissue mass, albumin, and hepatic blood flow associated with aging have been hypothesized to alter local anesthetic metabolism and elimination. While an increase in fatty tissues was observed to increase local anesthetic volume of distribution in one study with lidocaine , this finding was not supported by later studies examining intravenous lidocaine infusions [14, 15]. Similarly, while albumin levels decrease with aging, plasma protein binding of local anesthetics remains largely unchanged as alpha-1 acid glycoprotein levels are minimally altered [16]. Likewise, despite a 20–40% decrease in hepatic blood flow in geriatric patients, local anesthetic hepatic metabolism remains unaltered [17]. Consequently, local anesthetic metabolism remains largely unaltered with aging unless complicated by significant liver disease.
Potency and Onset
Local anesthetic potenc y is primarily due to lipid solubility. The aromatic ring and its substitutions and additions to the terminal amine determine lipid solubility. Specifically, the terminal amine may exist in a quaternary form (four bonds, positive charge, water soluble) or tertiary form (three bonds, neutral, lipid soluble). The ratio of drug’s solubility in a nonpolar solution (n-octanol) may be used to describe lipophilicity. This is known as the octanol-water partition coefficient , and the increased lipid solubility is associated with greater values [11, 18] (Table 19.1).
Local anesthetic onset is determined by the drug ionization constant (pKa). In order to stabilize local anesthetic bases in solution, clinical solutions are produced in a hydrochloride salt (pH 4–6). This converts them into a water-soluble (quaternary) state. Consequently, the onset is related to conversion to the tertiary (lipid soluble) form on exposure to physiologic pH (7.4). This conversion is determined by pKa, a pH that leads to 50% ionized and 50% non-ionized local anesthetic molecules. As local anesthetics are weak bases, their pKas are greater than 7.4. The greater the pKa of the drug, the greater the proportion in the quaternary form and the slower the onset.
Other factors can also impact the onset. Physiologic factors, such as increased tissue acidity caused by inflammation, can increase drug ionization and further delay quaternary to tertiary conversion. This is one explanation for the difficulty in anesthetizing infected tissue [12, 19]. The rate of diffusion can also be expedited by increased concentration. However, the relationship between onset and concentration is not linear but logarithmic. Therefore, doubling the concentration only marginally expedites block onset; however, it will provide denser blockade. This is commonly utilized with the ester local anesthetic chloroprocaine. Although it has a high pKa (8.9), it can be administered in high concentrations and high doses for rapid anesthesia onset. This is permissible due to its rapid metabolism by pseudocholinesterases. Local anesthetics are prepared in a range of concentrations to assist in the onset for less potent medications. For example, bupivacaine is very lipid soluble and therefore often utilized in lower concentrations (0.25–0.5%, 2.5–5 mg/ml) compared to lidocaine (1–2%; 10–20 mg/ml), which is less soluble.
Duration
Duration is generally described as short, intermediate, or long. Local anesthetic duration has classically thought to be directly related to protein binding. Factors keeping the drug near the nerve (increased lipid solubility, decreased tissue vascularity, presence of vasoconstrictors) may be of importance as well in prolonging duration of action.
In an effort to maximize onset and duration of the local anesthetics, it was often a common practice to combine short- and long-acting local anesthetics (e.g., mepivacaine and bupivacaine). However, evaluation of this practice revealed surprising results. When comparing interscalene blocks with mepivacaine, bupivacaine, or an equal mixture of each; the onset times for each group were nearly identical. Conversely, duration of the block was significantly decreased when compared to bupivacaine alone [20]. These results have been confirmed in other brachial plexus blocks [21]. Moreover, this effect was preserved whether local anesthetics were given as mixed or sequentially [22]. Therefore, if increased block duration is important, a mixture of different local anesthetics should be avoided. Conversely, mixing of nonlocal anesthetic perineural adjuncts (Table 19.2) has been described to increase anesthetic or analgesic duration. While epinephrine is the most common perineural additive, it has only been demonstrated to prolong the duration of short-acting local anesthetics (e.g., lidocaine ); however, it serves a valuable role as a marker for intravascular injection. Clonidine is another well-studied additive for both peripheral and neuraxial regional anesthesia techniques [24]. While clonidine is thought to act centrally through alpha-2 receptor stimulation, its peripheral mechanism is attributed to inhibition of the hyperpolarization-activated cation currents [26]. If clonidine doses exceed 100–150 mcg, sedation and hypotension become more common which may be of greater concern in the geriatric population [31]. While considered an accepted practice for both peripheral and neuraxial blockade, perineural clonidine remains an off-label use. Similarly, other adjuvants are considered off-label and experimental until further literature is available. With the success of clonidine, studies have recently examined dexmedetomidine, a more specific alpha-2 agonist, as a peripheral nerve block additive. Peripheral analgesic effects of dexmedetomidine are also attributed to inhibition of the hyperpolarization-activated cation currents [25]. The addition of 100μg of dexmedetomidine has been postulated to be the ideal dose. This has shown to nearly double the duration of interscalene nerve block and prolong supraclavicular nerve blocks [32, 33]. While dexmedetomidine has known side effects of sedation, hypotension, and bradycardia, when comparing IV and perineural dexmedetomidine to placebo, no difference in the incidence of significant adverse events was noted [34]. Similarly, dexamethasone has been reported to prolong brachial plexus blocks in numerous reports, with low perineural doses (1–2 mg) being as effective as higher doses (4–8 mg) [27]. Whether the improved analgesic outcome of dexamethasone is a local or systemic effect has been greatly debated. However, the studies comparing systemic and perineural administration have only examined large doses of dexamethasone approaching the systemic analgesic doses (0.1 mg/kg); this question continues to stimulate debate [28, 29]. Buprenorphine is another additive where it is unclear whether the mechanism of analgesia is systemic or peripheral when administered perineurally [23]. While buprenorphine’s use has shown improved analgesia in patients undergoing sciatic and axillary nerve blocks, its utility is limited by drug-associated nausea [23, 35]. Importantly, as neuronal toxicity is a concern with the use of perineural additives, basic science work has indicated that the most neurotoxic agent is often the local anesthetic [30]. Prolongation of local anesthetic duration can decrease the need for systemic analgesics which may be beneficial in elderly patients who are more susceptible to systemic opioids. While local adjuvants can help prolong local anesthetic duration, they are not without side effects, and the benefit of prolonged blockade should be weighed against possible adverse reactions.
Table 19.2
Summary of perineural additives examined in the literature
Medication | Mechanism of action | Potential concerns | Sites studied | Prolonged durationa | Dosing |
---|---|---|---|---|---|
Buprenorphine [23] | Debated | Pruritis | Axillary, sciatic | Variable | 0.3 mg |
Clonidine [24] | Inhibition of hyperpolarization-activated cation currents | Bradycardia, hypotension, sedation | Numerous | 2 h | 30–300 μg (often 150 μg) |
Dexmedetomidine [25] | Inhibition of hyperpolarization-activated cation currents [26] | Bradycardia, hypotension | Interscalene, axillary, posterior tibial | 4–5 h | 100 μg 150 μg 1 μg/kg |
Debated | Toxicity concerns with high doses [30] | Interscalene supraclavicular sciatic | 4–10 h | 1–10 mg | |
Epinephrine | Vasoconstriction | Potential neurotoxicity [30] | Numerous | 0 h | 2.5–5 μg/ml |
Minimum Effective Volumes
The increased popularity of ultrasound guidance has helped to decrease both the incidence of vascular puncture and the onset time of regional anesthesia [36]. It has also allowed for extremely precise deposition of local anesthesia and decreased local anesthetic volumes for a given regional block. This last point contrasts with nerve stimulation techniques in which nearly 40 ml of local anesthetic was often recommended to ensure block adequacy.
Decreasing the volume of local anesthetics administered can allow for a larger margin of safety and allow for multiple regional blocks while still remaining under a toxic dosage of local anesthetics. Most studies have shown a similar onset time for the lower volumes. While mixed results have been found for block duration, most decreases in volume result in a shorter duration.
Toxicity
Local anesthetic protein binding is concentration dependent and influenced by the pH of the plasma. As pH decreases, the percentage of bound drug decreases. As acidosis develops, as can occur with hypoventilation, seizures, or cardiac arrest, the percentage of free (unbound) local anesthetic increases. As the percentage of bound bupivacaine changes from 95 to 75%, the percentage of free drug is amplified fivefold (5–25%), although the total drug concentration remains the same. This increase in free local anesthetic drugs with acidosis renders bupivacaine distinctly toxic.
Local Anesthetic Systemic Toxicity (LAST )
Local anesthetics absorption into the systemic circulation may lead to local anesthetic systemic toxicity (LAST). Signs of LAST classically occur on a spectrum. The standard description of LAST begins with CNS excitation, tinnitus, agitation, metallic taste, or perioral numbness. This rapidly progresses to seizures and CNS depression. Neurologic signs are followed by cardiac manifestations beginning with hypertension and arrhythmias and escalate to conduction blockade, bradycardia, and asystole.
In the classic presentation of LAST, symptoms rapidly begin following the administration of local anesthetics, likely reflecting direct intravascular injection. Slower presentations can also manifest several minutes following local anesthetic administration secondary to delayed absorption or partial intravascular injection in the distal periphery. Because of the possibility of delayed presentation, the American Society of Regional Anesthesia (ASRA) suggests a minimum of 30 min of monitored care following administration of large doses of local anesthetics [37]. Despite the classic LAST manifestations, multiple symptoms can occur simultaneously or cardiac arrest can be the presenting sign.
Treatment of LAST depends on the presenting symptoms. ASRA has published a checklist to promote comprehensive treatment. In all scenarios, calling for help and lipid emulsion to the bedside should occur first as symptoms may rapidly progress. For the initial CNS signs, supportive care with prevention of hypoxemia and acidosis is the main goal. Should seizures ensue, symptoms must be rapidly controlled to prevent physical trauma and acidosis. Benzodiazepines are the preferred pharmacological treatment for seizure abolition because of the lack of cardiac depressant effects. While thiopental and propofol have been used, they should be considered second line drugs and slowly titrated to minimize cardiac depression. If cardiac symptoms develop, management of the airway to prevent hypoxemia and worsening acidosis can help to lessen LAST severity. This should be quickly followed by CPR and ACLS; however, epinephrine dosing should be decreased (1 μg/kg) and vasopressin, calcium channel blockers, and intravenous lidocaine should all be avoided. Lipid emulsion (20%) should be bolused (1.5 ml/kg) and then an infusion initiated (0.25 ml/kg/min). Additional boluses should be given in the setting of prolonged cardiovascular collapse and the infusion increased (0.5 ml/kg/min) if hypotension persists. The lipid infusion should be continued for a minimum of 10 minutes following return to cardiovascular stability [38]. These recommendations do not differ for the geriatric population.
Basic Principles and Blocks for Regional Anesthesia
Regional anesthesia may be used as the primary anesthetic or for postoperative pain management in the geriatric population. Patients receiving peripheral nerve blocks as the primary anesthetic have demonstrated decreased rates of postoperative sedation, nausea, and vomiting with improved pain control and expedited discharge times compared to patients receiving general anesthesia [39]. Further, regional anesthesia has been associated with decreased rates of chronic pain in certain surgical populations [40].
Both neuraxial and peripheral nerve blocks can be challenging in geriatric patients due to arthritis and decreased flexibility, leading to difficulty with positioning. While ultrasound guidance can ameliorate some positioning obstacles, ultrasound utilization is often greater with peripheral than neuraxial techniques.
Spinal and Epidural Blockade
Neuraxial anesthesia may be safely performed in geriatric patients; however, it can present challenges that are different compared to younger patients. As the body ages, the neuraxial anatomy degenerates, leading to multiple possible spinal abnormalities. Elderly patients develop osteoporosis, compression fractures, herniated disks, and many other changes at increased rates compared with younger cohorts. Degenerative changes can lead to loss of intervertebral height or rotational distortion of the spine. Spinal stenosis, an anatomic change leading to narrowing of the spinal canal, has an incidence of approximately 14% in patients at the age of 40 years. This incidence increases to nearly 40% as patients approach 60 years of age [41]. These anatomic changes, leading to decreased epidural space potential volume, may be partially responsible for increased dermatomal spread of epidural injectate. It has been shown that an equal dose and volume of local anesthetic will have a slower onset time in the elderly patient but will provide a higher block height when compared to middle-aged patients [42]. In fact, some advocate decreasing epidural medication volumes because of the increased segmental spread [43]. Calcification of spinal ligaments , also associated with aging, can lead to an inability to access the neuraxis via the midline, often requiring a paramedian approach [44]. Geriatric patients are also more likely to have had spinal surgery leading to epidural adhesions, scarring, gross anatomic modifications from instrumentation or bone grafting, and damage to spinal ligaments [45]. These anatomic changes can alter the spread of local anesthetics in the epidural space leading to patchy or inadequate blocks. Aging also leads to changes in CSF volume and production levels. The total CSF volume increases with age, mostly compensating for the loss of brain volume [46]. While there is an increase in volume, CSF production slows with increasing age [47].
Despite these anatomical changes, neuraxial anesthesia is commonly utilized in multiple geriatric surgeries including lower extremity orthopedic procedures and joint arthroplasty, urologic procedures, and certain vascular cases. As people continue to age, there is a known decline in parasympathetic activity which leads to predominant sympathetic tone; this leads to increased blood pressure lability, elevated dependence on preload, and decreased responsiveness to chronotropic and inotropic medications. These clinical changes in the autonomic nervous system may complicate hemodynamic stability regardless of anesthetic choice and should be monitored closely. In patients with hip fractures requiring surgical intervention, the superiority of regional anesthesia to general anesthesia for multiple postoperative outcomes ranging from postoperative delirium and decreased hospital stay to pneumonia and death is continuously debated [48, 49]. While studies continue, regional anesthesia is at least as safe as general anesthesia and may have multiple benefits for the geriatric hip fracture patient.
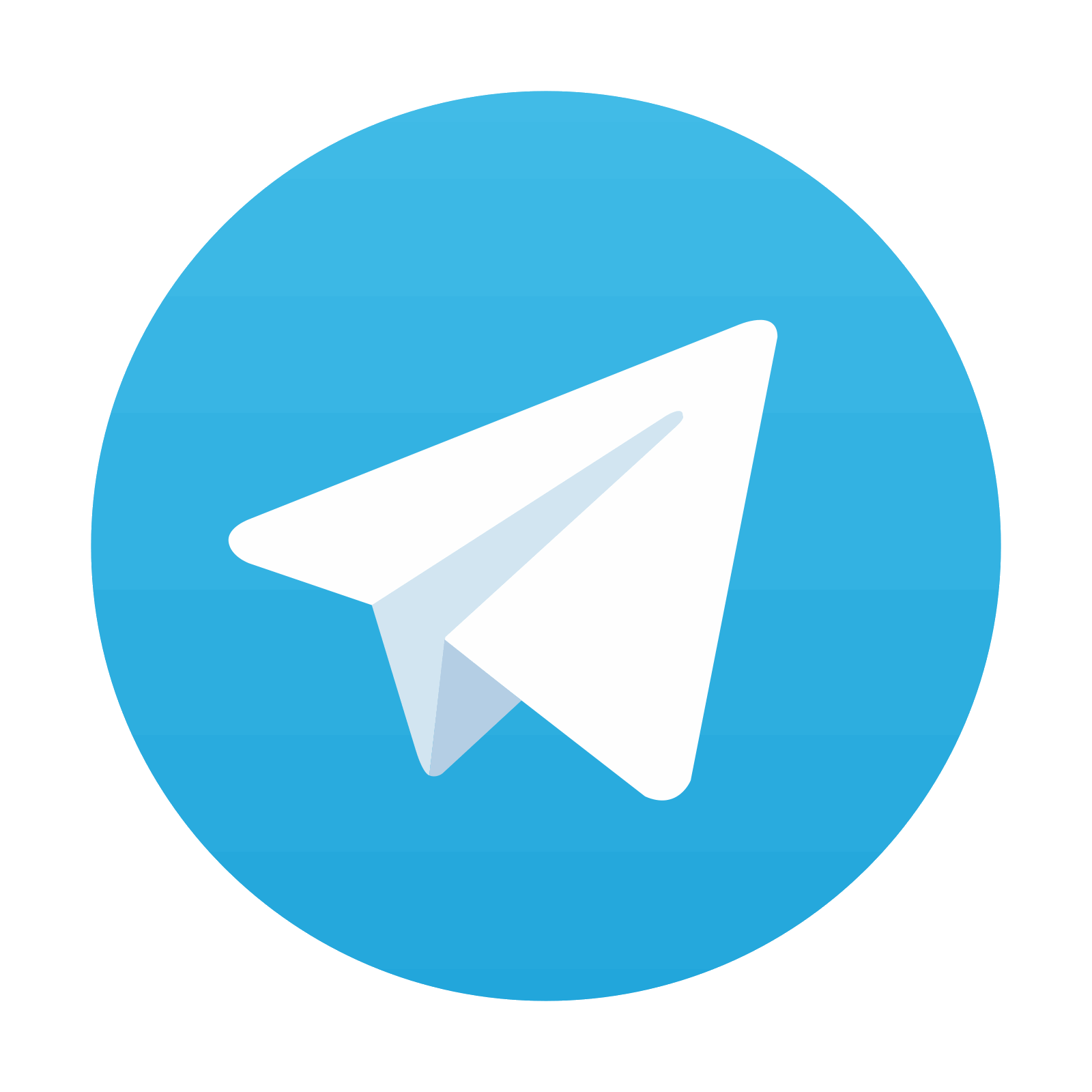
Stay updated, free articles. Join our Telegram channel
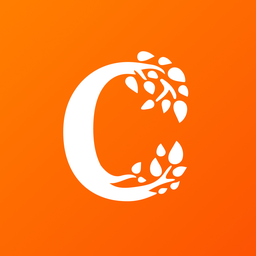
Full access? Get Clinical Tree
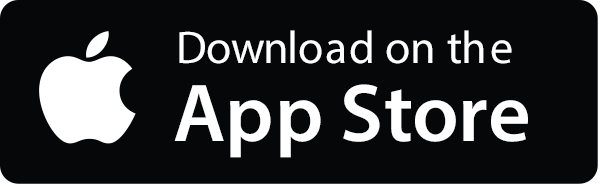
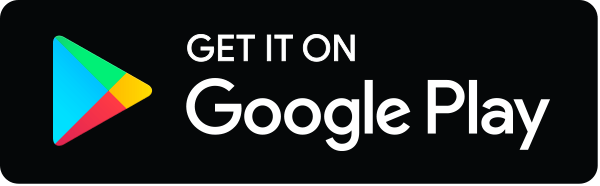