With each heart beat, the left ventricle moves a volume of blood into the systemic arterial vasculature for distribution to the organs of the body. This interaction between the left ventricle and the systemic arterial vasculature (ventricular-arterial coupling) is an important determinant of left ventricular function. Study of ventricular-arterial coupling once required invasive techniques. However, advances in echocardiography now make it possible to noninvasively assess ventricular-arterial coupling in humans. In this issue of JASE , Kuznetsova et al use echocardiographic techniques to better understand ventricular-arterial coupling and its effects on left ventricular performance in patients with hypertension. Such studies remind us of the core physiologic concepts pioneering cardiovascular scientists defined many years ago. As we enter an era of increasingly sophisticated noninvasive evaluation of cardiac performance, careful application of these core concepts is essential. These physiologic concepts form the basis for our understanding of the how the heart adapts to hemodynamic challenges during health and disease.
Early researchers worked with both isolated cardiac muscle and the intact heart, using the attributes of each preparation to their advantage. Study of isolated cardiac muscle allowed more strict control of experimental variables to clearly determine the effects of load on muscle function. Researchers found that adding increasing weight to isolated cardiac muscle before contraction results in greater force generation during contraction. These observations lead to the development of the concept of preload, the force stretching the muscle before the onset of contraction. Researchers also found that adding weight to isolated muscle after the onset of contraction, decreases muscle shortening as the weight increases. These observations lead to the concept of afterload, the load the muscle must bear after the onset of contraction. In general terms, diastolic filling pressure is widely used as the equivalent of preload while systolic arterial pressure is widely used as the equivalent of afterload in the intact heart. Research at an ultrastructural level found that as cardiac muscle is stretched by increasing preload there is an increasingly favorable overlap of the actin and myosin filaments resulting in increased active tension generation up to the point of maximal overlap, beyond which no additional active tension is generated. This length-tension research provided an understanding of the ultra structural basis of the Frank-Starling relationship and complemented the ongoing work in the intact heart. Early researchers also found that increased calcium availability to the contractile apparatus increased the rate and force of contraction. These effects on the rate and force of contraction were defined as a change in contractility, separate from the effects of preload and afterload on cardiac muscle.
Research using the intact heart by Frank, Starling, and others that followed showed that cardiac output is the product of stroke volume (end-diastolic volume minus end-systolic volume) and heart rate. From a simplistic point of view, end-diastolic volume is determined by atrial pressure and end-systolic volume determined by both arterial pressure, as well as by contractility. To better understand the effects of diastolic pressure on stroke volume independent of heart rate, the Frank-Starling relationship can be viewed as the filling pressure-stroke volume relationship for the ventricle. This allows us to more easily understand the effects of diastolic ventricular pressure on stroke volume. After accounting for external cardiac restraint, the filling pressure-stroke volume relationship for either the right or left ventricle is characterized by a relatively steep ascending limb and a relatively flat plateau limb. As filling pressure increases stroke volume increases up to a point. Once on the plateau limb of the filling pressure-stroke volume relationship, stroke volume increases very little as filling pressure increases. The filling pressure-stroke volume relationship of the ventricles relates primarily to ventricular diastole or end-diastolic volume.
An equally important but less often used relationship describes the effect of systolic pressure on end-systolic volume. The systolic pressure–end-systolic volume relationship is characterized by a relatively steep descending limb as aortic systolic pressure increases. With any increase in aortic pressure, end-systolic volume is larger with a smaller difference between end-diastolic volume and end-systolic volume resulting in a smaller stroke volume. Changes in myocardial contractility alter the position of both the filling pressure-stroke volume relationship and the aortic systolic pressure-stroke volume relationship with an increase in contractility shifting these relations up to a new relationship with a greater stroke volume at any given pressure.
As we use the term contractility it is important that we remember how contractility is defined. Contractility, an often misused term, defines the performance of the heart at a given preload and afterload. Left ventricular contractility is specifically defined as performance of the ventricle at a given filling pressure and systolic pressure. Myocardial contractility represents the intrinsic ability of the cardiac myocyte to contract independent of preload and afterload. Differences in the ability to produce force during contraction result from alterations in the amount of binding between myosin thick filaments and actin thin filaments. The degree of interaction between these filaments depends on the amount of calcium available for this process. Intrinsic or extrinsic activation of the myocardial beta-1 receptors or inhibition of myocardial phosphodiesterase makes more calcium available during systole to facilitate interaction between myosin and actin filaments; thus, increasing the force of contraction of the cardiac myocytes. With respect to the diastolic filling pressure-stroke volume relationship, end-diastolic pressure is the prime determinant of end-diastolic volume and stroke volume. However, an increase in contractility will shift the filling pressure-stroke volume relationship up and to the left while a decrease in contractility will shift this relationship down and to the right ( Figure 1 ). Acute changes in contractility and its effect on the position of the filling pressure-stroke volume relationship are due to changes in the availability of calcium to the contractile apparatus of the cardiac myocytes. More long-term changes in contractility can result from advantageous or disadvantageous remodeling of the ventricle.

Pressure-volume relationships can also be used to assess ventricular function throughout a series of cardiac cycles. The ventricular pressure-volume relationship can be plotted as a loop comprised of ventricular systole starting with the isovolumetric contraction phase, then the ejection phase followed by ventricular diastole with the “active” isovolumetric relaxation phase and ending with passive filling of the ventricle. Venous return and ascending aortic pressure can then be varied to generate a series of different pressure-volume loops ( Figure 2 ). Each pressure-volume loop describes a specific point determined by end-systolic pressure and end- systolic volume. As this specific end-systolic pressure-volume point varies with changes in preload and afterload, a relationship between these points can be determined. The slope of this relationship is defined as the end-systolic elastance (Ees) of the ventricle. Ees is a measure of ventricular performance that comprises myocardial contractility as well as geometric and structural components. Any short-term change in this relationship can reasonably be attributed to a change in contractility of the ventricle while long-term changes may reflect change in contractility or ventricular remodeling. Pressure volume loops can also be used to assess left ventricular work as the area enclosed by the left ventricular pressure volume loop. Alternatively, regional myocardial work can be measured noninvasively with strain Doppler echocardiography. This noninvasive method assesses regional myocardial work as the area enclosed in the pressure-myocardial strain loop during the ejection phase of the cardiac cycle.
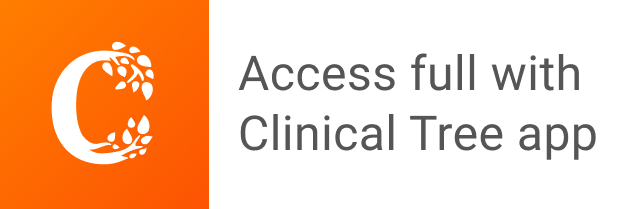