INTRODUCTION
In the era of classical cardiovascular (CV) medicine (ending around the year 2000), CV function was validated predominantly by invasive means. Classic studies established the end systolic and end diastolic pressure-volume relationships as a meaningful and useful way of characterizing intrinsic ventricular pump properties. These concepts then evolved such that they could be applied to basic and clinical research.
In the present era of CV intervention (projected to last until approximately 2020), CV imaging and molecular science are emphasized. Mathematical modeling and further clarification of diastolic function through the use of invasive and noninvasive technologies is evolving.
Around 2020, CV medicine is envisioned to evolve toward an “era of CV disease prevention.” Prevention will emphasize system physiology complemented by mathematical probability. A portfolio of physiologic models will be used as surrogate expressions of disease, and primary prevention of disease will be based on the intensity or burden of a physiologic or molecular/chemical risk model. Information acquisition will move from invasive to noninvasive.
Research and clinical physiologic approaches are currently evolving from invasive to noninvasive means to elucidate fundamental CV physiology. Clinical physiology is no longer limited to catheterization, and the noninvasive echocardiologist can be redefined as an “echophysiologist.”
This chapter attempts to relate classical invasive physiology to the current evolution toward Doppler echocardiographic physiology. It is increasingly recognized and emphasized that contraction (systolic function) is dependent on relaxation (diastolic function). It is important to appreciate the two distinct, although intimately interrelated, aspects of the assessment of cardiac properties: (1) of the ventricle as a hemodynamic pump and (2) of the intrinsic properties of the cardiac muscle. Systolic and diastolic ventricular properties are dependent on systolic and diastolic myocardial properties, as well as muscle mass, chamber architecture, and chamber geometry. Pressure-volume constructs are used to directly assess ventricular properties.
PATHOPHYSIOLOGY
Cardiac dysfunction, with or without systolic dysfunction, is associated with diastolic dysfunction (i.e., elevation of filling pressure). Cardiac dysfunction can be broadly viewed as a derivative of altered cardiac geometry and cardiomyocyte dysfunction or altered extracardiac loading, which is most commonly attributed to arterial stiffening. Once the sentinel cardiac or arterial perturbation is initiated, the dysfunctional state is propagated throughout the contiguous CV system (arteries, pump, and reservoir), becomes cyclical and self-perpetuating, and accounts for the clustering of a portfolio of common adverse events (e.g., heart failure [HF], atrial fibrillation, stroke, cognitive dysfunction).
Clinical Diastolic Dysfunction
Diastolic dysfunction refers to abnormal mechanical relaxation (diastolic) properties of the ventricle, which are present in the majority of patients with congestive heart failure (CHF). The term diastolic heart failure (DHF) is conventionally used to describe patients with CHF symptoms and normal systolic contractility (i.e., normal ejection fraction [EF]), although a preferred term would be HF associated with preserved systolic function. Thus, the distinction between DHF and diastolic dysfunction is merely the presence of CHF symptoms. The CHF symptom complex associated with systolic HF (SHF) or DHF is most strongly correlated with the elevation of filling pressure.
In order for the left ventricle to function as an effective pump, it must be able to empty and fill without requiring abnormal elevation of left atrial (LA) pressure. Atrial pressure is directly related to the ability of the left atrium to expel its contents into the ventricle during diastole. Thus, the principal determinant of risk and symptoms of CHF is the increased pressure reflected backward into the left atrium and pulmonary veins during diastolic filling of the ventricle. The stroke volume must be able to increase in response to stress, such as exercise, without any appreciable increase in LA pressure ( Fig. 7-1 ). Drugs that produce a sustained decrease in ventricular filling pressures enhance effort tolerance, breathing capacity, and HF outcome.

Pressure and Volume
Diastolic function conventionally has been assessed on the basis of the left ventricular (LV) diastolic pressure-volume relationship and an upward shift of the curve or increase in LV end diastolic pressure (LVEDP) ( Figs. 7-2 and 7-3 ). The LV end diastolic pressure-volume relationship is the most important means of characterizing global filling properties. The pressure-volume loop (see Fig. 7-2 ) depicts the amount of diastolic filling (ml of blood) relative to a specified filling pressure (mmHg) and is therefore a key physiologic determinant of preload. Any delay or impedance of myocardial relaxation will increase the filling pressure, which will be reflected backward into the left atrium and pulmonary veins.


The end diastolic pressure-volume relationship is intrinsically nonlinear (see Fig. 7-3 ), a characteristic attributed to the different types of structural fibers being stretched in different pressure-volume ranges. In the low pressure-volume range, with only a small increase in pressure for a given increment in volume, the stretch of compliant elastin fibers and of the myocytes with sarcomeric titin molecules is believed to account for stiffness. As volume is increased to a higher range, pressure rises more steeply as slack lengths of collagen fibers and titin are exceeded and stretch is more strongly resisted by these stiff elements. Therefore, chamber stiffness increases as end diastolic pressure or volume increases.
With diastolic dysfunction, any abnormal increase in diastolic filling pressure corresponds to a less distensible or less compliant ventricle during the filling phase of the cardiac cycle. Consequently, a “stiff” ventricle is less able to increase its stroke volume without further elevation of LA pressure. Pressure reflected backward through the open mitral valve into the atrium and pulmonary veins can cause shortness of breath, elevation of pulmonary venous pressure, secondary pulmonary hypertension, and decrease in clinical exercise capacity.
INVASIVE VERSUS NONINVASIVE ASSESSMENT
Systolic Function
Cardiac function assessment traditionally has focused on decreased contractility and the measure of EF. The noninvasive measure of systolic function, EF, is almost exclusively used in clinical trials and medical practice. Although EF is clinically considered an important index of LV systolic function, it is not exclusively governed by LV properties. Rather, EF is determined by the interaction of arterial and ventricular properties. A normal EF suggests that contractility and arterial stiffness are well matched. EF is very load dependent and normally increases up to 30% during stress. EF is thus limited by many factors, including marked preload dependence and low reproducibility when measured by different imaging modalities. Furthermore, EF greater than 45% does not contribute to assessment of CV risks in patients with HF. In patients with CHF and elevated filling pressure, diastolic dysfunction correlates better with B-type natriuretic peptde concentration and mortality than with EF or LV volume.
Diastolic Function
Although the invasive research “testing ground” has made important contributions to the understanding of pressure-volume relationships and diastolic physiology, only recently has assessment of diastolic function been extensively incorporated into clinical practice. Assessment of diastolic function was neglected because there was no reliable noninvasive method to assess diastolic dysfunction, and invasive confirmation of elevated filling pressure was deemed impractical if not unethical. Invasive monitoring through a pulmonary artery catheter or implantable transducer can provide continuous data. Pulmonary artery catheters, however, appear to contribute to morbidity and mortality and contribute little to clinical assessment. Even when LV cardiac catheterization is performed, the principal measure of diastolic function is LVEDP, which measures only the load-dependent filling pressure and reflects conditional physiologic circumstances only at the time of data acquisition.
With the advent of noninvasive Doppler echocardiography, clinical assessment of diastolic function has become increasingly attainable and validated. However, early impediments to clinical use of Doppler echocardiographic diastolic function assessment became apparent. The first measures of diastolic function, which relied on mitral and pulmonary vein flow, were load dependent—similar to EF and LVEDP—and changed dynamically even during the examination. Because of the dynamic nature of these physiologic events, measures of diastolic function were limited for use in the assessment of long-term morbidity and mortality.
STATE-OF-THE-ART INVASIVE AND NONINVASIVE PHYSIOLOGY
Diastolic function assessment by both invasive and noninvasive means is plagued by ambiguities surrounding the dynamic nature, indirectness, and difficulty of reproducibly measuring ever-changing diastolic function. Dynamic measures include:
- 1.
Decrease in pressure during the period of isovolumic relaxation (invasive: time constants of relaxation; noninvasive: deceleration time and longitudinal fiber relaxation)
- 2.
Passive and active chamber stiffness or, its inverse, compliance (invasive: elastance; noninvasive: diastolic grade and LA volume)
- 3.
End diastolic pressure (invasive: LVEDP; noninvasive: Doppler E/E′ ratio, end diastolic mitral versus pulmonary vein Doppler flow) ( Table 7-1 )
TABLE 7-1
DIASTOLIC FUNCTION ASSESSMENT BY INVASIVE AND NONINVASIVE MEANS
METHOD
Functional parameter
Invasive
Noninvasive ultrasonography
Myocardial relaxation
Mathematical models of pressure-volume loops
Tissue Doppler annular velocity, E′
Ventricular and arterial stiffness
Analysis of viscoelastic properties : simultaneous measurement of pressure, volume, and flow
Vascular: pulse pressure, central blood pressure
Measures of “stiffness” : effective arterial elastance (E a ), end systolic elastance (E es ); Doppler-derived diastolic dysfunction.
Vascular stiffness : pulse wave velocity, augmentation index
Filling pressure
Left ventricular end diastolic pressure
Doppler E/E′ : acute load-dependent filling pressure
Left atrial volume : chronic average increase in filling pressure
Myocardial Relaxation
Invasive Assessment
Pressure relaxation can be measured invasively by placing a micromanometer catheter into the left ventricle. The rate of pressure decrease, which begins during the isovolumic period, is influenced by several physiologic factors :
- 1.
Capacity and rapidity with which the sarcoplasmic reticulum and sarcolemmal Na + /Ca 2+ exchanger restores cellular Ca 2+ levels to low diastolic levels
- 2.
Extent of elastic recoil (suction) within the heart caused by compression by myocyte contraction during the prior systole and history of prior systolic ejection, which is largely determined by the aortic input impedance (stiffness)
- 3.
Other chamber-level factors, such as dyscoordination and heterogeneity
Isovolumic pressure relaxation traditionally starts when pressure decay is maximal, generally occurring shortly after aortic valve closure, and extends to a point when LV pressure decreases just below a threshold equal to the end diastolic pressure plus 5 mmHg. Recorded pressure-time curves can be mathematically analyzed to extract the rate of pressure decrease. However, the type of model used to describe relaxation can have marked influences on the apparent changes with drug and chamber-loading interventions. Model-dependent analysis of relaxation is commonly fraught with systematic deviations from the real data, particularly with depressed cardiac function. Some diseases, including hypertrophic cardiomyopathy, are characterized by regional heterogeneity of loading and material properties, which result in nonuniform relaxation. Diseases with a complex process of pressure decrease, such as hypertrophic cardiomyopathy, in general cannot be easily expressed in a single time constant.
Noninvasive Assessment
Diastolic dysfunction is associated with substantial abnormalities of active relaxation and passive stiffness of the ventricle. Doppler transmitral velocity deceleration time (DT) and tissue Doppler early diastolic mitral annular velocity (E′) are commonly used noninvasive indices of myocardial relaxation. DT fluctuates with the dynamic changes in ventricular loading pressure, whereas E′ is relatively load independent. DT is useful for estimating intensity or “grade” of dysfunction at the time of the examination. Tissue Doppler E′ velocity is touted as less load dependent and can distinguish pseudonormal from normal filling patterns. The pseudonormal filling pattern is commonly encountered when using mitral inflow Doppler to characterize diastolic function grade.
Active (e.g., Ca 2+ -dependent) and passive (e.g., fibrosis) structural mechanisms regulate the rates of ventricular pressure decay and early diastolic ventricular filling. Active relaxation represents the speed of transition from the contracted state (systole) to the relaxed state (diastole) and is strongly related to the uptake of calcium for the contracted myocyte. Passive relaxation is most strongly related to myocardial compliance and the effects of tissue fibrosis. Load-dependent measures reflect the dynamic physiologic state of filling pressure under the conditions existing at the time of the examination as well as the likelihood of a favorable response to a therapeutic manipulation (modifiability). The tissue Doppler E′ velocity reflects the relaxation velocity of the myocardium and expresses both passive and active myocardial relaxation. Variations in long-axis shortening have relatively little effect on the EF or stroke volume. Most of the stroke volume is produced by shortening of the minor axis dimension, which explains why either decreased diastolic (E′) or decreased systolic annular tissue Doppler can be observed in the presence of a normal EF.
Ventricular Stiffness
Invasive Assessment
Ideally, to analyze the viscoelastic properties of the ventricle, one should take simultaneous measurements of chamber pressure, volume, and flow. Passive elastic properties are those that relate a change in pressure to a given change in volume, whereas viscous properties are those that relate change in pressure to the rate of change in volume or flow. As opposed to noninvasive Doppler, no invasive methods are readily applicable for measuring flow rates of filling in the human heart. Therefore, most analyses must obtain flow data from the derivative of volume. The invasive measurement of pressure uses a high-fidelity micromanometer catheter. Measurement of volume is more challenging, with investigators commonly using various imaging-based methods to construct a volume-time curve. This approach is labor intensive, typically yielding a limited number of samplings of volumes during the diastolic period, and is subject to various sources of noise given the extensive processing required. A notable alternative is intracardiac electrical impedance, which can serve as a continuous measure of chamber volume.
The association between diastolic pressure and volume from a single cardiac cycle does not necessarily follow a simple mathematic relationship. Only limited filling property data can be obtained from a single cardiac cycle. Various solutions have been used to overcome these limitations. After a diastolic pressure-volume curve is obtained, these data are typically subjected to a mathematical curve-fitting process to extract parameters of chamber or muscle stiffness. As with relaxation analysis, there are important limitations of such fitting when applied to a diastolic curve, owing to the nature of the mathematics. Unless the diastolic data are perfectly monoexponential, which is rarely the case, simply evaluating the same curve or restricting analysis to slightly different ranges of volume and pressure can influence the derived parameters. Ultimately, derived stiffness parameters must be viewed cautiously, particularly if the data themselves do not clearly follow an exponential rise waveform. Even if directly measured by invasive pressures and volumes, the relationship between the two variables during filling does not necessarily provide information solely related to the cardiac chamber.
Noninvasive Assessment
Limited success has been achieved in replicating pressure-volume loops by noninvasive Doppler echocardiography. However, several noninvasive studies have identified a relationship between limitation of exercise capacity, increased CV stiffness, and DHF. Noninvasive Doppler echocardiographic assessment of “stiffness” appears to be an excellent means of expressing ventricular and arterial stiffening. Effective arterial elastance (E a ), which reflects vascular stiffening, and end systolic elastance (E es ), which reflects ventricular stiffening, can be calculated noninvasively and validated against invasive assessment ( Fig. 7-4 ). E a , E es , and diastolic stiffness increase with age and correlate with one another. Normally, there is an inverse relationship between E es and E a . As arterial compliance decreases (increased stiffness), ventricular compliance would be expected to increase (decreased stiffness). In order for EF to increase during exercise, the coupling ratio of E a and E es must decrease. However, with aging, the increase in exercise EF becomes blunted, suggesting age-associated differences in the coupling ratio (E a /E es ) and its components. Both arterial and ventricular stiffness tend to increase to a similar degree—E a /E es remains constant despite an increase in arterial stiffness (see Fig. 7-4 ). Thus, ventricular stiffness tends to increase with age.

As ventricular and arterial stiffness increase, the system becomes more pressure labile and sensitive to loading conditions. This physiologic change is most evident with aging, as reflected in increased systolic and pulse pressure after age 50 to 60 years. These changes explain decreased exertional capacity and pressure-loading amplification associated with age. Diastolic HF with preserved EF appears to be best correlated with increased ventricular-arterial stiffness. Relaxation of the “stiff” ventricular myocardium is delayed when the ventricle is exposed to increased systolic pressure during ejection (i.e., increased afterload). Diastolic dysfunction, as reflected in increased filling pressure, is directly related to greater prolongation of diastolic relaxation and increased ventricular-vascular stiffening.
A major hemodynamic consequence of arterial stiffening is widening of the arterial pulse pressure, which also increases cyclic changes in arterial flow. Increased arterial stiffness increases systolic pressure and decreases diastolic pressure. Coronary perfusion occurs principally during diastole; thus, in persons with stiff conduit vessels and lower diastolic pressure, coronary microvascular perfusion is adversely affected. Impaired myocyte relaxation of the subendocardial longitudinal fibers is an age-associated finding. Reduced myocardial relaxation is best measured as a decrease in tissue Doppler E′ velocity.
Structural remodeling and functional disturbances are essential to the development of diastolic dysfunction and HF, but acute or subacute decompensation with CHF generally requires a precipitant, or “trigger.” The stiff arterial system is particularly sensitive to acute dysfunction, as occurs with excessive sodium intake, hypertension, atrial fibrillation, myocardial infarction, or marked bradycardia (prolonged diastasis). High arterial pulsatility is noted to have detrimental effects on blood flow regulation and contributes to adverse mechanical forces, which affect vascular tone, atherogenesis, angiogenesis, hemostasis, and microvascular autoregulation.
Filling Pressures
Invasive Assessment
LVEDP is the most common measure of diastolic dysfunction recorded during clinical cardiac catheterization. Increased filling pressure is not a requisite of diastolic dysfunction but is a universal feature of both systolic and diastolic dysfunction. Increased filling pressure is not specifically representative of either systolic or diastolic dysfunction but is a potent marker of evolution toward overt CHF and hospitalization. Diastolic filling pressure reflects the load-dependent changes in chamber stiffness, with diastolic dysfunction uniformly occurring before or coincident with overt systolic dysfunction. The decrease in cyclic adenosine monophosphate needed to impair diastolic relaxation is much smaller than is required to impair contractility. Abnormal diastolic dysfunction is thus an earlier and more sensitive marker of a deficient energy state in HF than are abnormalities of systolic performance. For example, if EF is greater than 45%, it does not further contribute to assessment of CV risk in patients with HF. The principal determinant of HF risk is the inability of the ventricle to properly fill during diastole (i.e., inability to “prime the pump”).
Noninvasive Assessment
The easiest and most reproducible noninvasive measure of filling pressure elevation is the ratio of early diastolic transmitral inflow velocity (E) to E′ (Doppler E/E′ ratio). The E/E′ ratio has been well correlated with invasive measures of LV filling pressure and pulmonary capillary wedge pressure. The ratio depicts the relationship between dynamic changes in LA pressure and LV compliance. As LA pressure increases, E increases, depicting the increased pressure gradient between atrium and ventricle. Conversely, myocardial relaxation, as depicted by tissue Doppler E′ velocity of the mitral annulus, is unchanging and relatively unaffected by loading dynamics. The E/E′ ratio, which is load dependent, reflects filling pressure at the time of the recording. An E/E′ ratio greater than 10 is highly sensitive (92%) and specific (80%) for the prediction of pulmonary wedge pressure greater than 15 mmHg. Filling pressure is dynamic; thus, it is common to record a normal filling pressure at rest and an elevated filling pressure with exercise. E/E′ should be viewed as a measure of the intensity of the filling pressure abnormality at the time of recording.
Increased LA size reflects the average burden of volume or pressure overload or both. Atrial volume index is the preferred means of expressing atrial size because the atria enlarge asymmetrically and in direct relationship to the body surface area. Atrial enlargement because of abnormal pressure overload is substantiated by the coexistence of abnormal tissue relaxation (i.e., low E′ velocity) ( Fig. 7-5 ); conversely, atrial enlargement because of benign volume overload is associated with a normal Doppler E′ velocity. LA volume index increases slowly and changes little with acute pressure or volume overload. Indexing LA volume to body surface area accounts for plasma volume throughout life.

LA volume index best reflects “chronicity,” or average filling pressure, and the E/E′ ratio best reflects the “intensity” of filling pressure elevation at the time of the recording. For example, persons with chronic, yet intermittent, elevation of filling pressure (increased LA volume index and abnormal E′) can have a normal E/E′ at rest and an abnormal E/E′ with exertion. Clinical outcome is more strongly related to the average burden of filling pressure elevation as reflected in the atrial volume and not the resting grade of diastolic dysfunction.
Comprehensive Diastolic Function Assessment
The pressure-volume loop and tissue Doppler echocardiography both demonstrate the close link between systolic and diastolic function. Patients with compensated systolic dysfunction have lesser degrees of diastolic dysfunction. Conversely, patients with overt CHF have higher grades of diastolic dysfunction. Diastolic dysfunction is not a part of “normative aging” but an age-related risk associated with the development of increased total CV risk, including increased HF, atrial fibrillation, and mortality, independent of age and sex.
As advances in noninvasive methods continue to evolve, reliance on invasive methods will continue to decrease. The invasive examination remains the physiologic testing ground for understanding instantaneous physiology. However, the Doppler echocardiographic examination, although not ideal, is now the gold standard for clinical assessment of diastolic function and physiologic CV burden, in addition to complementing CV research.
NORMAL DIASTOLIC MYOCARDIAL PHYSIOLOGY
During LV contraction, potential energy is normally stored as the myocytes are compressed and the elastic elements in the LV wall are compressed and twisted. Relaxation of the myocardial contraction allows this potential energy to be released as the elastic elements recoil. The release of wall tension (potential energy) is normally rapid enough to cause the LV pressure to decrease despite an increase in LV volume (see Fig. 7-1 ). Although there is a relative decrease in early diastolic enhanced LV relaxation and elastic recoil from tachycardia and adrenergic stimulation during exercise, LA pressure does not increase to an abnormal level. Neither an increase in atrial pressure nor more vigorous atrial contraction contributes to the increased mitral valve pressure gradient and resulting increase in dV/dt max that occurs during exercise. The intraventricular pressure gradient is greater in normal subjects than in patients with HF. In order for LV contraction to release the store of elastic potential energy, LV volume must decrease below a critical value during contraction. The minimum end systolic volume required to generate suction equals the equilibrium volume, the volume of the fully relaxed ventricle at zero pressure. If either the equilibrium volume decreases or the end systolic volume increases, the ventricle will be unable to release potential energy capable of suctioning blood into the ventricle. Other determinants of suction are the time constant of LV relaxation and the degree of large-scale LV torsion and twist.
Temporal-Spatial Pressure and Flow: Suction of Blood into the Left Ventricle
Peak flow across the mitral valve normally equals or exceeds the peak flow rate across the aortic valve. The rapid movement of blood from the left atrium into the left ventricle in early diastole is due to a pressure gradient from the left atrium all the way to the LV apex ( Fig. 7-6A ). Ventricular filling is thus primed by a rapidly developing pressure envelope extending between the left atrium (highest pressure) and the LV apex (lowest pressure). As a consequence of the early diastolic intraventricular gradient between the left atrium and ventricular apex, blood is virtually “sucked” into the left ventricle. Suction is initiated during isovolumic ventricular relaxation and continues during early rapid filling. The lower the early diastolic LV pressure, the greater the suction, which allows the heart to function at lower LA pressure. Diastolic suction contributes to filling more than one order of magnitude greater than does passive atrial decompression.

Left Atrial Pressure and Left Ventricular Suction
The ability to dynamically decrease early LV filling pressure is particularly important in response to stress, which allows an increase in LV stroke volume without an appreciable increase in LA pressure. Diastolic intraventricular pressure gradients are the result of the interaction of inertial (local) and convective forces caused by unsteady intracardiac flow and pressure distribution. Inertial forces are caused by the impulse (potential pressure) developed by myocardial restoring forces related to the inotropic state (LV contraction and the creation of potential energy). Convective deceleration is determined by spatial-temporal filling flow velocity and, most particularly, the presence of a normal cone-shaped ventricular geometry (see Fig. 7-6A ).
When the mitral valve opens and blood is normally sucked into the left ventricle, the gradient between that ventricle and the left atrium begins to decrease, and flow ultimately stops or transiently reverses when these pressures equilibrate. Under normal circumstances, relaxation is completed during rapid filling as the LV pressure attains its near minimum. More than two thirds of the LV stroke volume normally enters the left ventricle during this earliest phase of diastole. The time of pressure deceleration is determined by normal vigorous suction (active force) or by “stiff” noncompliant LV muscle (passive force) associated with disease states. The normal response to stress is an increase in the gradient between the left atrium and the LV apex. β-adrenergic stimulation increases contractility, myocardial restoring forces, and the resulting enhanced ventricular suction. Increased diastolic suction facilitates rapid filling and lowers minimum LV pressure. Enhanced diastolic suction acts as a compensatory mechanism to maintain low pulmonary venous and arterial pressure in situations of increased contractility.
The gradient between the left atrium and the LV apex is determined by inertial acceleration (elastic recoil) and convective deceleration (chamber geometry) of blood. Inertial acceleration is the change in velocity with respect to time, and convective deceleration is proportional to the decrease in velocity with respect to distance. Convective deceleration is increased when the distance or architecture of the left ventricle is altered and intraventricular blood flow is diverted away from the longitudinal axis of the left ventricle. An increase in convective deceleration caused by altered ventricular geometry thus decreases the normal gradient between the left atrium and the LV apex (see Fig. 7-6 ).
Late in diastole, atrial contraction produces a second LA-to-LV pressure gradient that again propels blood into the left ventricle. After atrial systole, as the left atrium relaxes, its pressure drops below LV pressure, which initiates closure of the mitral valve. The onset of ventricular systole produces a rapid increase in LV pressure that seals the mitral valve and ends diastole.
CLINICAL RELEVANCE
A thorough understanding of normal and abnormal myocardial physiology is vital to the determination of cause and effect. CHF and the clustering of associated risk factors cannot be assumed to represent cause and effect. Although risk factors modify and enhance the severity of the physiologic condition, most risk events, such as CHF, atrial fibrillation, and stroke, are best viewed as consequences and not causes. In particular, age-associated CV disease appears to have a common physiologic basis, which best accounts for the clustering of common adverse events.
Epidemic of Diastolic Heart Failure
As the world’s population has increased, the average life expectancy has also increased by approximately 3 months per year since 1840. This fact best accounts for the existence of an artifactual age-associated global epidemic of CV risk. HF is the most common indication for hospitalization of older persons. However, it is also well known that at least 50% of patients with CHF have a normal EF, and two studies report that more than 70% of older patients with symptomatic HF have a normal EF and fit the diagnosis of DHF. In addition, more than half of patients with CHF are relatively asymptomatic, which contributes to a substantial underestimation of the true incidence of HF, particularly in older persons. However, patients with HF and preserved EF (i.e., DHF) have exercise intolerance, shortness of breath on exertion, episodes of pulmonary edema requiring hospitalization, and increased mortality, similar to patients with SHF. In general, mortality with CHF is approximately 50% at 5 years. Patients with DHF tend to be older and more likely to have a history of hypertension, atrial fibrillation, and fewer symptoms, whereas patients with SHF are more likely to have a longer history of CHF, ventricular arrhythmias, and clinical manifestations of coronary artery disease.
Clinical and research emphasis is increasingly placed on the physiology of cardiac dysfunction and not merely systolic contractility (i.e., EF). State-of-the-art noninvasive Doppler echocardiographic physiology has become the standard of clinical and investigative assessment of CV function. Thus, any discussion of CV function must incorporate the strengths and unique attributes of invasive and noninvasive cardiac and vascular physiology used in the assessment and understanding of diastolic and systolic function.
Symptoms of CHF are strongly related to the elevation of filling pressure, which accounts for breathlessness, exercise intolerance, and reduced quality of life. CV dysfunction leading to elevation of filling pressure and the clustering of adverse events can be broadly grouped as a consequence of either altered cardiomyocyte function accompanied by distorted ventricular geometry or abnormal cardiac loading, which most commonly begins as a consequence of extracardiac stiffening of the conduit arterial system (see Fig. 7-2 ).
Diastolic disorders from cardiomyocyte dysfunction include dilated cardiomyopathy, hypertrophic cardiomyopathy, and ischemic heart disease. Diastolic disorders beginning with increased pressure loading are most commonly ascribed to increased pulse pressure and central systolic blood pressure (i.e., hypertension).
Energy-dependent myocardial relaxation (diastolic function) is metabolically more vulnerable than systolic contraction. Resistance to ventricular filling reflects pressure backward into the atria and the pulmonary veins during diastole when the atria and ventricles are directly exposed to each other. As the volume in the atrium and pulmonary vein increases, their combined reservoir capacity is ultimately exceeded. The dynamics of the left atrium and pulmonary veins undergo a transition from physiologic volume overload to abnormal pressure overload (see Fig. 7-5 ). The transition from a state of benign atrial volume overload to pressure overload is commonly slow and intermittent. The transition to a pressure overload state is clinically more apparent with stress intolerance and acute congestive symptoms after a stressful trigger (e.g., illness, surgery, infarction). Stress-induced increase in atrial pressure accounts for breathlessness and exercise intolerance. Sustained increase in pulmonary venous pressure accounts for symptoms ascribed to the syndrome of CHF.
Myocardial Disease and Diastolic Dysfunction
Abnormal suction of blood into the left ventricle has been replicated with various HF models, such as myocardial ischemia and hypertrophic cardiomyopathy (see Fig. 7-6B ). Any condition that interferes with normal regional systolic function might be expected to modify the pattern of the normal early diastolic intraventricular pressure gradients. With LV muscle disease, the end diastolic pressure-volume curve is shifted substantially to the right, and the diastolic pressure curve is shifted upward, reflecting the increase in filling pressure (see Fig. 7-3B ). Abnormal LV filling dynamics are seen, along with increased LA pressure and inability to increase stroke volume without abnormal elevation of LA pressure. In patients with dilated cardiomyopathy, the normal pressure gradient from the left atrium to the LV apex is substantially decreased. Hypertrophic cardiomyopathy is a myocardial disease with abnormal ventricular geometry and preserved systolic function and is also accompanied predominantly by abnormal diastolic function.
Diastolic suction is disturbed because of abnormal changes in chamber geometry, which are commonly associated with uncoordinated wall motion or impaired myocardial contractility. The decreased capacity to generate suction contributes to the abnormal increase in filling pressure. Limited suction response to stress causes LV filling pressure to increase disproportionately and causes exercise-related shortness of breath. Decreased suction shifts the pressure-volume curve toward a higher minimum diastolic pressure (see Fig. 7-3B ). With dobutamine-induced stress, the gradient between the left atrium and the LV apex can be demonstrated to be impaired. Altered geometry of the ventricle impairs inertial acceleration (decreased global elastic recoil) and enhances convective deceleration. Myocardial disease, associated with impaired elastic recoil and enhanced convective deceleration, is proportional to the magnitude of altered LV geometry. Cardiomyopathic ventricles show abnormal suction at baseline and have a limited ability to recruit suction when undergoing inotropic stimulation. The minimum diastolic pressure of the failing heart increases during exercise.
Although the increase in filling pressure accounts for the symptoms of CHF (i.e., increased filling pressure and decreased EF), it is not directly related to the hemodynamic cause of decreased cardiac output and distorted LV geometry. Thus, simply decreasing filling pressure will decrease symptoms but will fall short of eliminating the primary problem. Lasting clinical improvement requires reversal of the LV remodeling and return of the cardiac output and renal perfusion to near-normal values to prevent relapse of fluid retention and subsequent clinical deterioration.
Arterial Stiffening and Diastolic Dysfunction
Widening of the arterial pulse is common to aging and generally reflects the stiffening or senescence of the conduit arteries and a dominant hemodynamic risk for cardiac dysfunction. In response to an increase in preload volume, the increase in systolic and diastolic blood pressure is exaggerated, which accounts for the shortness of breath, exercise intolerance, and hypertensive pulmonary edema seen in patients with DHF. The blood vessels are coupled in tandem with the ventricle (pump), which undergoes simultaneous systolic and diastolic stiffening (see Figs. 7-3 and 7-6C ). Combined stiffening alters how the heart-arterial system interacts at rest, but particularly under stress by exertional demands, and is particularly evident in patients with CHF and preserved EF, smaller-than-normal end diastolic volume, and normal ventricular geometry. Delayed active and passive relaxation of the stiff left ventricle in early diastole disturbs the normal suction gradient between the left ventricle and the left atrium. HF with preserved EF and a normal-sized and -shaped left ventricle more commonly occurs in older persons, women, and obese persons and is associated with a lower rate of myocardial infarction.
Vascular stiffness affects CV reserve, coronary and peripheral flow regulation, endothelial function, and mechanical signaling and causes blood pressure lability and diastolic dysfunction. Age-associated ventricular systolic and diastolic stiffness occurs even in the absence of other CV disease and is thought to be the dominant cause of age-related HF with preserved EF. Stiffening of arterial-ventricular function is referred to as coupling disease and is considered to be the principal contributor to the epidemic of age-associated CV adverse events such as HF, atrial fibrillation, stroke, and cognitive dysfunction.
Physiologic Coupling of the Cardiovascular System
Reservoir, Pump, and Arteries
The concept of “continuity disease” has been proposed to describe the relationship and shared physiology between the arteries and the rest of the CV system. The CV system is best viewed as a contiguous system, analogous to a conventional water pump. The heart is a pump delivering pulsatile flow to the arterial system. The arterial and capillary vascular system is an extensive hydraulic filter, which converts pulsatile flow to continuous flow at the end organ. The atria and veins act as a distensible reservoir that stores blood during ventricular contraction and fills the ventricle during diastole, thus initiating the conversion of continuous flow back to pulsatile flow. Any abnormality that interferes with efficient forward-directed flow initiates deterioration in the forward and backward directions to the adjoining components of the contiguous system.
The age-associated epidemic of HF appears to be principally related to an increase in arterial stiffness. Increased arterial stiffness affects the ventricle (reverse direction) and distal arterial bed (forward direction). The concept of coupling disease can be expanded to include the interdependence of the whole CV system (i.e., arteries, pump, and reservoir).
Arterial-Ventricular Coupling
It is necessary to address the interaction of the whole CV system as a discipline in order to understand the vascular and cardiac regulatory mechanisms associated with the contiguous relationship of ventricular and arterial function. The interaction of ventricular and arterial properties, or coupling, is an important and largely underappreciated determinant of cardiac performance. Elastance is the change in pressure for a given change in volume of the ventricle. Arterial elastance (E a ) indexed to body surface (E aI ) represents a steady-state arterial property that characterizes the functional properties of the arterial system (see Fig. 7-4 ). E a is a lumped parameter that accounts for aortic impedance, peripheral resistance, and arterial compliance. E a is equal to the LV end systolic pressure divided by the stroke volume. E a shares common units with elastance measures of ventricular function (E es or E es indexed to body surface [E esI ]); their ratio (E aI /E esI ), an index of arterial-ventricular coupling, is inversely related to EF. In healthy subjects free of CV disease, EF increases by up to 30% during exercise. However, in order for the EF to increase during exercise, the coupling ratio, E aI /E esI , must decrease ( Fig. 7-7 ). Studies show that with aging, the normal increase in EF during exercise (i.e., EF reserve) becomes blunted, suggesting age-associated differences in the shift of coupling ratio and its components during exercise. Suboptimal vascular-ventricular coupling helps explain the age-associated blunting of maximal exercise EF and its underlying mechanisms.
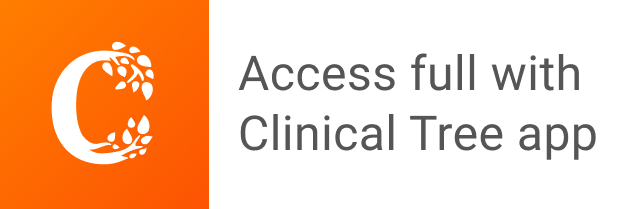