Dilip S. Nath1 and Richard A. Jonas2 1St. Louis Children’s Hospital, St. Louis, MO, USA 2Children’s National Hospital, Washington, DC, USA Interrupted aortic arch (IAA) is a rare anatomic finding that accounts for approximately 1.5% of all congenital heart anomalies [1, 2]. Recent developments in genetics and developmental biology have enhanced our understanding of the role of apoptosis, which causes embryonic resorption of most of the original six branchial arches. However, when apoptosis occurs in excess it may lead to IAA [3, 4]. Single‐stage surgical repair is now accepted as the standard of care in nearly all cases and can be achieved with low early surgical mortality. Continued efforts to mitigate the need for late reintervention, particularly for left ventricular outflow tract (LVOT) obstruction, have led to preoperative approaches to identify this challenging subset of patients as well as implement surgical strategies such as two‐stage (palliation followed by complete repair) techniques. The important association of IAA with chromosomal abnormalities, in particular microdeletion of chromosome 22, is critically important in the short‐term (surgical outcomes) as well as long‐term (quality of life, cognitive development) metrics. As stem cells, neural crest cells (NCC) are multipotent and are generated between the neural tube and nonneural ectoderm. They migrate from the neural tube after generation into embryonic areas where they differentiate into diverse cell types. During vertebrate development NCCs are responsible for craniofacial skeleton formation, pharyngeal arch artery remodeling, and septation of the cardiac outflow tract. Cardiac NCCs migrate to pharyngeal arch arteries and the cardiac outflow tracts through arches 3 to 6 form great vessel smooth muscle cells, and are involved with pharyngeal arch arteries and outflow tract remodeling [5]. Emphasis on identifying candidate genes that lead to IAA has resulted in a deeper understanding of the relevant molecular mechanisms, particularly the integral role of micro RNA (miRNA) in regulating gene expression. In the case of IAA, disturbance of NCC migration into derivatives of pharyngeal arches and pouches accounts for many developmental defects. RNase II endonuclease (Dicer) activity, which is important in generating functional miRNAs, can affect cranial and cardiovascular systems differently [6]. In cranial systems Dicer and miRNA are needed for survival, while in cardiovascular systems they are needed for migration and patterning of cells, whose absence can lead to IAA type B, double‐outlet right ventricle (DORV), and ventricular septal defect (VSD). Similarly, mice with NCC inactivation of Dicer die in the perinatal period [7]. Migration of cardiac or cranial NCC to tissue is less affected by Dicer being disrupted as opposed to its subsequent development. In summary, Dicer is needed for craniofacial development, morphogenesis of pharyngeal arch arteries, and its disruption (via the effect on the fourth pharyngeal arch artery remodeling) that can lead to IAA type B. Furthermore, Cdc42 (cell division cycle 42; Rho family small GTP‐binding protein) functions as a molecular switch to regulate cytoskeleton remodeling and cell polarity establishment [8]. In mice, inactivation of Cdc42 in NCC leads to embryonic death with craniofacial deformities and cardiovascular developmental defects, while playing an implicating role in NCC migration. Epigenetics is an important part of the development of the cardiac outflow tracts, particularly with respect to how histone deacetylases – Hdac3 (class I Hdac) – functions during mouse cardiac NCC development [9]. Loss of Hdac3 causes IAA type B, aortic arch hypoplasia, DORV, and VSD. It regulates smooth muscle differentiation in NCC and subsequent aortic arch arteries; class I Hdacs are regulators of transcription via chromatin‐mediated transcriptional repression and direct deacetylation of certain transcriptional regulators [10, 11]. β1 integrin (whose deletion is usually lethal prior to birth) is not essential for migration and initiation of differentiation of smooth muscle, but is critical for remodeling of pharyngeal arch arteries and assembly of vessel wall with their derivatives [12]. Approximately 1 out of 8 infants with congenital heart disease (CHD) can have a chromosomal abnormality, based on a recent analysis of 4500 infants (live births and fetal deaths) with CHD over 12 years from a population‐based surveillance system [13]. The most common CHD lesions identified were IAA (type B or otherwise; 69%), atrioventricular septal defect (AVSD; 67%), and DORV (33%). The most common chromosomal abnormalities noted were trisomy 21 (53%), trisomy 18 (13%), 22q11.2 deletion (12%), and trisomy 13 (6%). Deletion of chromosome 22q11.2 is a common chromosomal cause of CHD and has a prevalence of 1 out of 4000 live births [14, 15]; it causes CHD in 75% of cases, mainly abnormalities of the outflow tracts [16, 17]. Among IAA subsets, type B is most likely to have chromosome 22q11.2 deletion, while type A is less likely [18]. T‐box gene TBX1 (among the 30 genes deleted) is critical for many aspects of DiGeorge syndrome phenotype [19, 20]. The most common screening techniques include polymerase chain reaction with analysis of restriction fragment length [21]. Fluorescence in situ hybridization is the gold standard for detecting chromosome 22q11.2 deletion syndrome; however, multiplex ligation‐dependent probe amplification is preferred, because it is more economical and time efficient [22, 23]. Recent studies have focused on a chromosomal disorder known as cat‐eye syndrome, where the diagnosis is made by the presence of a small supernumerary marker chromosome (sSMMC) derived from chromosome 22 [24, 25]. The marker has been narrowed to the proximal segment of 22q11 [26]. Type B IAA and hydrocephalus were found in mosaicism of 1.37 MB amplified cat‐eye syndrome critical region. Cat‐eye syndrome can have phenotypic variations, including IAA and intracranial cysts. Children with right‐sided IAA type A and AVSDs have also been reported [27, 28]. In a large clinical study of over 1600 patients with outflow tract defects, the investigators found chromosome 22q11.2 deletion to be present in 48% of patients with IAA, and 35% of patients with common arterial trunk [29]. Early identification aids in family counseling and permits early intervention, given that 6% of childhood cases are inherited in an autosomal dominant fashion [30]. Clinical assessment alone is insufficient to predict chromosomal abnormality in patients with cardiac malformations; chromosome 22q11.2 deletion would be undiagnosed in up to 25% of patients if chromosomal testing were done solely by clinical examination [31]. De novo interstitial deletions in chromosome 5 (7.59 Mb deletion 5q23.1–q23.3) and chromosome 18 (7.34 Mb deletion 18q12.1–q12.3) have been identified in a fetus with a prenatal diagnosis (fetal demise) of IAA and atrial septal defect (ASD). Fluid from amniocentesis was used to perform whole‐genome aCGH (micro‐array based comparative genomic hybridization analysis) on uncultured aminocytes [32]. De novo mosaic PTPN12 partial deletion in a child with IAA type A, VSD, and pyloric stenosis has also been reported [33]. PTPN12 is a downstream target of the RAS pathway, which is involved with endothelial cell adhesion and migration. PTPN12 has an important role in cardiovascular development and vessel formation during embryonic development. Partial deletion of PTPN12 leads to IAA type A, possibly as a result of a null mutation in the RAS pathway. Mutations in Hox (Homeobox) gene (HoxA1 are transcriptional regulators) cause human syndromes with severe cardiovascular malformations [34–37]. In mice studies, HoxA1‐null mice developed IAA, aberrant subclavian artery, and tetralogy of Fallot. This demonstrates the importance of HoxA1 in patterning the great arteries and the outflow tract [38]. HoxA1 is present in precursors of cardiac NCCs and interacts with genes critical for neural crest specification. The extrapericardial component of the ascending aorta normally gives rise to the brachiocephalic or “innominate” artery as its first branch. This artery usually branches to give rise to the right common carotid and right subclavian arteries. The aortic arch itself commences distal to the origin of the brachiocephalic artery, and has proximal, intermediate, and distal components (Figure 14.1). The proximal arch extends from the takeoff of the brachiocephalic artery to the left common carotid artery. The intermediate component extends from the left common carotid artery to the takeoff of the left subclavian artery and can be termed the “distal arch.” The most distal segment of the arch terminates at the juxtaductal region of the descending aorta, and is termed the isthmus [39]. These segments of the arch have different embryologic origins. The ascending component of the extrapericardial aorta together with the proximal segment of the arch are remnants of the aortic sac, which is the manifold interposed between the distal component of the intrapericardial outflow tract and the origin of the arteries running through the pharyngeal arches (Figure 14.2). The intermediate and distal components of the arch are formed from the artery that runs through the left‐sided fourth pharyngeal arch. The left subclavian artery, which is the seventh cervical intersegmental artery, is initially positioned caudal to the junction between the artery of the left‐sided sixth arch and the descending aorta. With ongoing development, the artery migrates cranially, crossing the junction between the artery of the left‐sided sixth arch and the descending aorta. The length of the isthmus then depends on the extent of cranial migration, with the artery of the left‐sided sixth arch persisting to form the arterial duct [40]. During normal development, the initially symmetric arteries running through the pharyngeal arches undergo significant apoptosis. Because of these apoptotic activities, it is only the left‐sided pathways that normally persist (Figure 14.2). It is not unreasonable, therefore, to postulate that apoptotic changes can also remove developing segments of the left‐sided arch, thus producing interruptions between its developmental components. Figure 14.1 Components of the aortic arch. The proximal component refers to the “proximal arch” (C), which extends from the takeoff of the brachiocephalic artery to the left common carotid artery (LCC). The intermediate component refers to the “distal arch” (B), which extends from the LCC to the takeoff of the left subclavian artery (LSA). The distal component refers to the “isthmus” (A), which extends from the most distal segment of the arch and terminates at the juxtaductal region of the descending aorta. RCC, right common carotid; RSA, right subclavian artery. Source: Reproduced by permission from Jonas RA, in Pediatric Cardiac Surgery, 4th ed., Oxford: Wiley; 2013, pp. 283–294. The classification usually used to distinguish the different sites of interruption (Figure 14.3) was introduced in 1959 [2]. The so‐called type A interruption, the second most common arrangement, occurs at the level of the isthmus. There is usually a gap between the two segments of the aortic arch. It can be found nonetheless in a milder form, in which a short fibrous cord connects the blind‐ending intermediate segment of the arch supplying the left subclavian artery with the descending aorta, in the absence of luminal continuity. In both variants, the descending aorta itself is supplied through the persistently patent arterial duct. The arrangement with the fibrous cord is well described as atresia of the isthmus. Taken together, the variants with isthmic interruption or atresia account for the nearly 25% of cases coming to surgical correction. The so‐called type B variant is found when the arch is interrupted between the origins of the left common carotid and left subclavian arteries, with the left subclavian artery and the descending aorta then supplied through the persistently patent arterial duct. This pattern accounts for nearly 70% of cases [41–43]. It is often associated with an aberrant origin of the right subclavian artery from the descending aorta. This means that during fetal development there is more flow of blood through the persistently patent arterial duct, and less through components of the arch fed from the LVOT than when the right subclavian artery retains its normal origin from the brachiocephalic artery. It is not surprising, therefore, that subaortic stenosis is found more frequently when the right subclavian artery arises aberrantly in retroesophageal fashion. The so‐called type C interruption is the rarest variant, occurring in less than 5% of cases [42]. It represents interruption between the carotid arteries, with the left common carotid and subclavian arteries depending on ductal patency for their vascular supply. Although the distal segment of the interrupted arch is usually fed through the persistently patent arterial duct, it is possible to encounter patients in whom the duct has undergone spontaneous closure and become ligamentous. In these patients, the flow to the distal segments is through collateral vascular channels. Apart from the persistently patent arterial duct found in almost all cases, a VSD is the most commonly associated anomaly and is found in nearly 75% of patients [42]. The defect typically produces obstruction to the LVOT because of posterior malalignment of the muscular or fibrous outlet septum (Figure 14.4). The flow to the proximal component of the interrupted arch can be reduced because of hypoplasia of the orifice of the aortic valve, which is frequently bicuspid with commissural fusion. Obstruction can also be produced by hypertrophy of a prominent muscle bundle on the left ventricular free wall that projects into the outflow tract, the so‐called muscle of Moulaert (Kreutzer J and Jonas R, unpublished observations). It is most unusual to find a fibrous subaortic shelf producing obstruction in the neonate with an interrupted arch, but this lesion can develop within a year or two of repair. The atrial septum is frequently deficient in the setting of an interrupted arch, usually because of stretching of the patent foramen ovale. This finding can permit significant interatrial shunting, and hence achieve hemodynamic importance. It is likely the consequence of the left‐to‐right shunting produced during fetal development because of the left‐sided obstruction, which results from the interruption combined with the frequent obstruction of the LVOT. Figure 14.2 Reconstructions of the developing arteries running through the pharyngeal arches in humans (upper panels) and mice (lower panels). The upper left‐hand panel, from a human embryo at Carnegie stage 14 (equivalent to around six weeks of development), shows that the arch arteries in the frontal projection are symmetric at this stage, arising from the aortic sac. The numbers show the arteries of the third, fourth, and sixth arches. The upper right‐hand panel, from a human embryo at Carnegie stage 17 (equivalent to around seven weeks of development), shows how the upper arms of the aortic sac have remolded to become the brachiocephalic artery and the intermediate component of the transverse aortic arch, with the left fourth arch becoming the distal component of the definitive arch. The distal outflow tract has separated to become the intrapericardial components of the arterial trunks. At this stage, the seventh cervical segmental artery has still to migrate to reach its definitive location; it is not possible to recognize the isthmus. The left sixth arch has become the arterial duct. The lower left‐hand panel shows a developing normal mouse subsequent to formation of the definitive aortic arch, and the lower right‐hand panel shows a mouse in which the Tbx1 gene has been knocked out, producing so‐called “type B” interruption, with the arch interrupted between the origins of the left common carotid and subclavian arteries. The reconstructions were prepared by Dr. Simon Bamforth, from the Institute of Genetic Medicine, Newcastle University, UK. We are grateful to Dr. Bamforth for permitting us to reproduce the images, which are based on his own research. Source: Reproduced by permission from Bamforth SD, Anderson RH. Cardiol Young. 2017;27(2):369–372. Figure 14.3 Anatomic types of interrupted aortic arch. (A) Type A, interruption distal to the left subclavian artery. (B) Type B, interruption between the left subclavian and left carotid arteries. (C) Type C, interruption between the left carotid and innominate artery. Ao, aorta; DA, arterial duct; IA, innominate artery; LCCA, left common carotid artery; LSA, left subclavian artery; MPA, main pulmonary artery; Prx Desc Ao, proximal descending aorta. Source: Reproduced by permission from Jonas RA, in Pediatric Cardiac Surgery, 3rd ed., Philadelphia, PA: Mosby; 2003, pp. 273–282. Figure 14.4 Interrupted aortic arch with ventricular septal defect in hearts with concordant ventriculo‐arterial connections. Pathological specimens showing (A) posterior deviation of the fibrous outlet septum and (B) posterior deviation of the muscular outlet septum. Although found most frequently with concordant ventriculo‐arterial connections, interruption of the aortic arch can also be found with DORV, common arterial trunk, or discordant ventriculo‐arterial connections. In the setting of double outlet or transposition, it is frequent to find subaortic obstruction due to narrowing of a completely muscular subaortic infundibulum, which in these settings represents obstruction to the right rather than the LVOT (Figure 14.5A). A similar mechanism can also be found in the setting of dominant left ventricle with incomplete right ventricle and discordant ventriculo‐arterial connections. In the latter setting the obstruction to aortic flow is typically found at the level of the VSD (Figure 14.5B). Figure 14.5 Interrupted aortic arch with transposition and ventricular septal defect. (A) Narrowed subaortic infundibulum and (B) double‐inlet left ventricle with a restrictive ventricular septal defect between the dominant left ventricle and the incomplete and rudimentary right ventricle, with the latter ventricle supporting the aorta. The incidence of IAA is 3 per million live births and it is commonly associated with patent arterial duct, bicuspid aortic valve, and isolated VSD [44]. A VSD is present in nearly 75% of patients with IAA [1]. An ASD is frequently seen in conjunction with IAA, usually in the form of a stretched patent foramen ovale. IAA is also seen in patients who ultimately undergo various forms of functionally univentricular palliation (3%), and in patients with a concomitant diagnosis of common arterial trunk (11%). Prenatal diagnosis of CHD by ultrasound is becoming increasingly common. IAA is a difficult diagnosis to make in fetal screening based on a standard four‐chamber view. To be definitive, transverse and longitudinal views of the upper mediastinum with a three‐vessel view of the aortic arch is needed. For screening, a low aortopulmonary diameter ratio in the presence of equally sized ventricles, aberrant right subclavian artery, or four‐dimensional echocardiogram may be more helpful to indicate IAA. Grayscale and color Doppler interrogation has helped to increase detection; nonetheless, only 10–40% of fetuses can be consistently diagnosed for IAA at most centers [45–47]. Isolated reports from very experienced centers have documented postnatal confirmation of fetal diagnosis of IAA with good accuracy [48]. As an adjunct to prenatal evaluation, postnatal pulse oximetry screening is increasingly used in nurseries to identify CHD. A recent study emphasizes that such screening may not be as relevant in a center where a robust fetal program exists, and hence most children with CHD are prenatally diagnosed. For example, over a 12‐month period in a quaternary care, newborn nursery pulse oximetry was performed in all newborns over 35 weeks’ gestation. Of these neonates, 99.5% passed the first screening, one infant failed three times and was diagnosed with pulmonary hypertension by echocardiography, and one child died but was later shown to have IAA [49]. This is not surprising given that while 25% of patients with critical CHD have coarctation of the aorta (CoA) or IAA, these lesions (in contrast to cyanotic ones) are not typically targeted for identification [50]. Measurement of upper‐ and lower‐extremity blood pressures is not without its challenges. Upper/lower‐extremity blood pressure gradient is a poor screening test with low sensitivity. In a recent large case–control study, neonates with unrepaired CoA/IAA had pressure gradients that were statistically higher than matched cohorts, but there was sufficient overlap between the cohorts leading to low sensitivity [51]. While blood pressure increases with gestational age, upper/lower‐extremity blood pressure gradient does not. Difference between upper/lower mean pressures can be attributed to random variability or to the same cuff being used to measure for both [52]. Accuracy may improve with four‐extremity blood pressure measurement at 24–48 hours following birth (after presumably duct closure) along with pulse oximetry screening. For the patient who is not diagnosed prenatally with the most common form of IAA (IAA/VSD), there is often low suspicion of serious heart disease during the early neonatal period until ductal closure begins. Prostaglandin (PGE1) is started immediately after the diagnosis is confirmed and an acidotic insult is avoided. Regression of both the right and left fourth aortic arches during embryonic development leads to different anatomic variants of IAA, depending on where the site of regression occurs [2]. After birth, if ductal closure should occur abruptly or is not recognized rapidly, the child will soon become profoundly acidotic and anuric, as perfusion of the lower body becomes entirely dependent on collateral communications between the two separate aortic systems. The distribution of palpable pulses will depend on the anatomic subtype. For example, with the common type B IAA, the right arm pulse will be palpable when the left arm and femoral pulses become impalpable secondary to ductal closure. Ischemic injury to the liver will be reflected in a marked elevation of hepatic enzymes (serum glutamic‐oxaloacetic transaminase [SGOT], lactate dehydrogenase [LDH]) and ischemic injury to the gut may be followed by evidence of necrotizing enterocolitis such as bloody stools. Renal injury is typically quantitated by the elevation observed in serum creatinine. A severe degree of systemic acidosis (prolonged pH less than 7.0) will ultimately result in injury to all tissues of the body. The child may have seizures, become flaccid, and respond poorly. Myocardial injury will be manifest as a low cardiac output state despite normalization of parameters. Since pulmonary blood flow is preserved during ductal closure, it is unusual to see pulmonary insufficiency. Occasionally the duct will not close during the neonatal period, and diagnosis may be delayed for several weeks. As pulmonary resistance falls there will be an increasing left‐to‐right shunt and the child will present with evidence of congestive heart failure, including failure to thrive. The first description of IAA was in 1778, with the first successful surgical repair (type A IAA) completed in 1957, before anatomic classification was fully described in 1959 [2, 53, 54]. Although it was considered to be fatal without surgical treatment within the first month, development of PGE in 1976 enabled children to be resuscitated before undergoing repair [55, 56]. One‐stage repair, which included addressing the commonly associated VSD, was first accomplished by Barratt‐Boyes using synthetic conduit to achieve arch continuity [57]. One‐stage repair including direct arch anastomosis was first described by Trusler in 1975 and is now the most common approach to repairing IAA/VSD [58]. With appropriate resuscitation of the neonate, IAA outcomes have improved dramatically over the past few decades. Accurate anatomic diagnosis can currently be made using echocardiography alone. In addition to localizing the site of the interruption, an echocardiogram should assess (i) length of the discontinuity, (ii) narrowest dimension of the LVOT (generally secondary to posterior displacement of the outlet septum), (iii) diameter of the aortic annulus, (iv) diameter of the ascending aorta, and (v) size/apex‐forming nature of the left ventricle. The features of associated anomalies must be carefully defined. For example, the location of an associated VSD should be defined in relation to its margins. The outlet septum is often severely hypoplastic, rendering approach to the superior margin of the defect through the tricuspid valve particularly difficult. Additional VSDs are very rare. Magnetic resonance imaging/angiography (MRI/MRA) is emerging as a useful adjunct to echocardiography [59]. For uncomplicated cases of IAA/VSD it has little advantage in the unrepaired neonate over echocardiography alone [60]. If there are important associated anomalies or unusual features, such as vascular rings in association with IAA, MRI/MRA can assist in surgical planning [61, 62]. Cardiac catheterization is of limited value, as the pertinent question of left ventricular adequacy is unlikely to be answered with hemodynamic measurements in the setting of ductal patency (with PGE) and, when present, the unrestrictive nature of VSD. PGE1 must be infused through a secure intravenous line, typically an umbilical venous catheter or a peripherally inserted central catheter (PICC) line. Establishing ductal patency is the first step in medically resuscitating the neonate with IAA. Given that the lower half of the body is dependent on perfusion through the ductus and blood in the ductus may pass into the pulmonary circulation, it is important that pulmonary resistance is maximized. This can be achieved by avoiding a high level of inspired oxygen, nitric oxide, or hyperventilation‐mediated respiratory alkalosis. Control of ventilation is best achieved by intubating the child and using sedation and paralytics. A peak inspiratory pressure and ventilatory rate should be selected to achieve a pC02 level of 40–50 mm. Myocardial function is likely to be depressed and, particularly given that the heart may need to handle a moderate volume load, an inotropic agent such as dopamine should be routinely employed. It is common for the neonate to require up to two days of medical resuscitation before surgery is undertaken. It would be very unusual for a child to be taken to the operating room with any abnormalities of acid–base, renal, or hepatic indices [41]. There is widespread agreement that primary one‐stage repair in the neonatal period is optimal management in nearly all instances. A staged procedure is employed when the child presents in shock and end‐organ failure, which has not been alleviated despite optimal medical management, and has associated lesions (most commonly substrate for LVOT obstruction), which may prompt some centers to avoid a neonatal complete repair strategy. When primary one‐stage repair is not performed, an initial palliative approach with application of bilateral pulmonary artery bands and maintenance of ductal patency with stenting or continued PGE infusion should be undertaken. Complete repair consisting of arch reconstruction, pulmonary artery debanding/reconstruction, and closure of the associated VSD is typically performed during infancy. During transport to the operating room and while preparing and positioning the child, it is important to avoid a high level of inspired oxygen and hyperventilation. It is preferable to place a right radial arterial line in addition to an umbilical arterial line. This will allow the team to (i) monitor perfusion of the separate upper‐ and lower‐body circulations during the cooling phase on cardiopulmonary bypass (CPB); and (ii) assess any pressure gradient across the anastomosis following repair. Approach is via a median sternotomy. Pericardium is harvested for VSD/ASD patch closure. Accurate arterial cannulation is an essential key to the success of the procedure. Generally an 8F or 6F arterial cannula is used for the ascending aorta. The cannula should be inserted on the right lateral aspect of the ascending aorta, exactly opposite the anticipated location of the arch anastomosis. The second arterial cannula is connected to the arterial tubing by a Y connector and is inserted on the anterior surface of the distal duct. Immediately after beginning bypass it is necessary to tie a suture ligature (typically 5‐0 polypropylene) around the proximal duct. Venous cannulation is accomplished via either (i) a single straight cannula in the right atrium for a child who weighs less than 2.5 kg, or if the approach for VSD closure is through the pulmonary artery; or (ii) bicaval cannulae for a child who weighs more than 2.5 kg, or if the approach for VSD closure is through the right atrium. During cooling the ascending aorta and its branches, ducts, and descending aorta are thoroughly mobilized. If an aberrant right subclavian artery is present, it should be ligated and divided at its origin from the descending aorta. It is often useful to divide the left subclavian artery in a type B IAA to further minimize anastomotic tension. When both rectal and tympanic temperatures are less than 18 °C, bypass is discontinued. Cardioplegia is infused through a sidearm on the ascending arterial connector, with temporary occlusion of the distal ascending aorta with forceps. Both arterial cannulae are then removed. The duct is ligated and divided at its junction with the descending aorta. Any residual ductal tissue is excised from the descending aorta. A C‐clamp is applied across the descending aorta to bring it closer to the ascending aorta so that the anastomosis can be performed under no tension. The anastomosis is sited completely on the ascending aorta and exactly opposite the ascending aortic cannulation site (Figure 14.6).
CHAPTER 14
Interrupted Aortic Arch
Developmental Biology
Genetics
Chromosome 22q11.2 Deletion
Other Chromosomal Deletions
Anatomy and Pathology
Associated Anomalies
Pathophysiology and Clinical Features
Prenatal Diagnosis
Management
Historical Perspective
Diagnosis of Interrupted Aortic Arch
Management of Interrupted Aortic Arch
Preoperative Management
Surgical Approach
Standard Surgical Technique: IAA/VSD
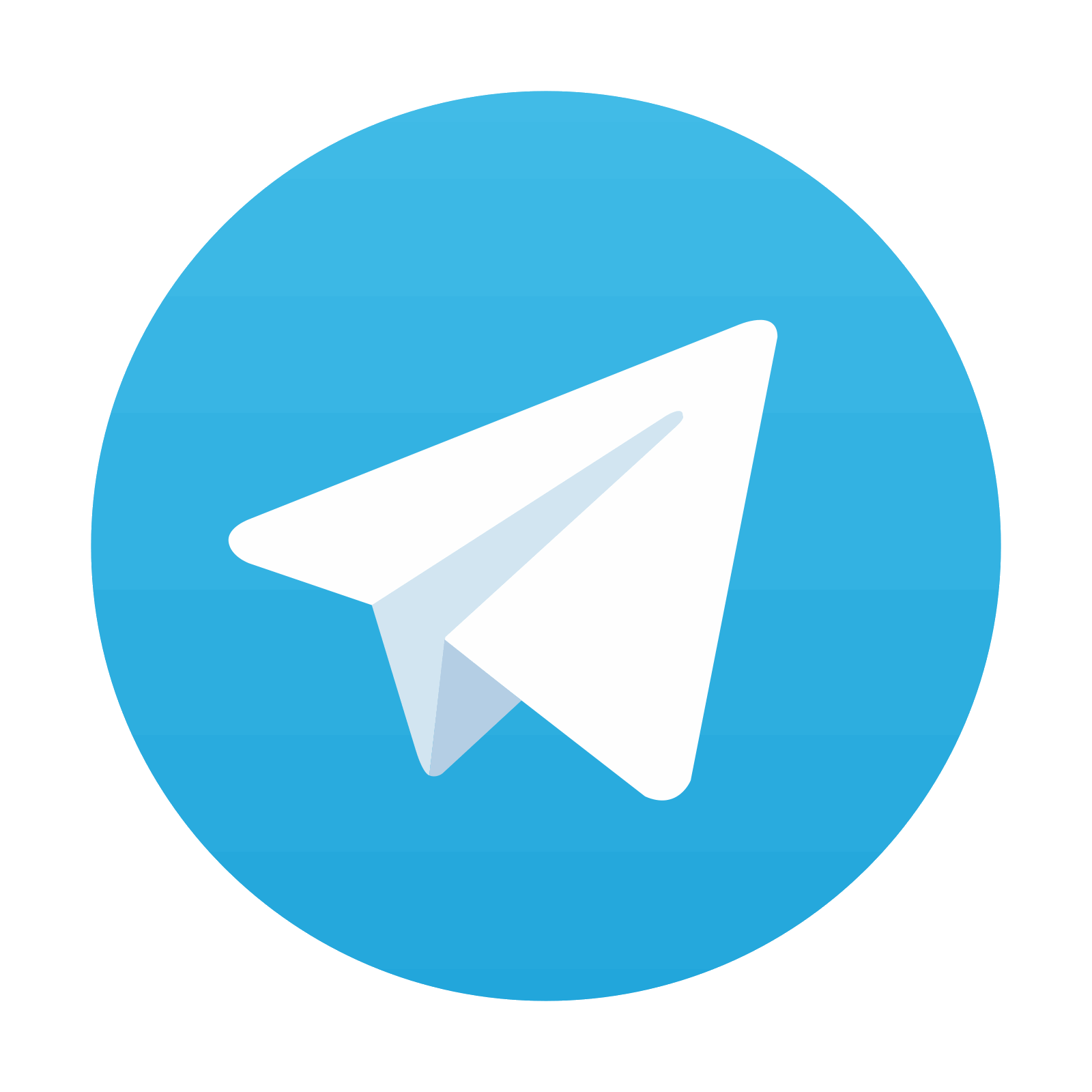
Stay updated, free articles. Join our Telegram channel
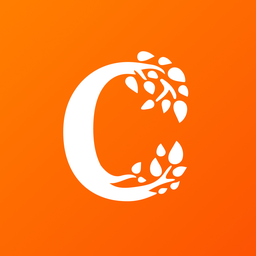
Full access? Get Clinical Tree
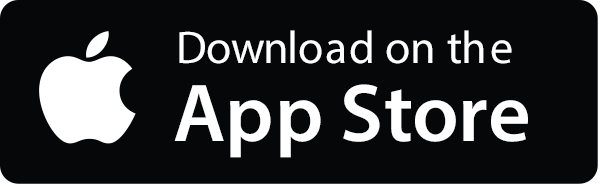
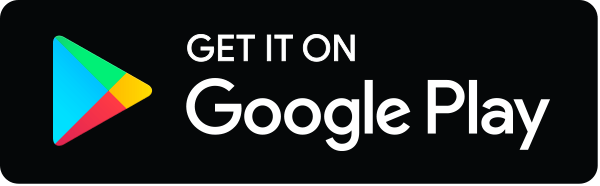