(A) Changes in FRC of rabbit pups (30 days) treated with either an epithelial sodium channel blocker (amiloride; filled circles) or saline (open circles) and mechanically ventilated from birth relative to mechanical breath number on the x-axis. No difference in FRC was observed between the amiloride and saline-treated groups. (B) Rate of change in FRC during the expiratory phase, in rabbit pups (30 days) ventilated from birth and treated with either an epithelial sodium channel blocker (amiloride; filled circles) or saline (open circles); a negative value indicates a net reduction in FRC. The decrease in FRC was significantly greater in the amiloride-treated group.
Airway Liquid Clearance After Birth
Phase-contrast X-ray image sequences and simultaneous plethysmography of spontaneously breathing term newborn rabbits at birth demonstrate that lung liquid clearance occurs during inspiration (36,39). The increase in transpulmonary hydrostatic pressure gradients generated during inspiration are likely responsible for the majority of airway liquid clearance at birth. The X-ray movie sequences clearly show that the air/liquid interface only moves distally toward the terminal airways during inspiration and, although some proximal movement can occur during expiration, little or no distal movement occurs between breaths (36,39) (Figure 9-2). Thus, liquid moves from the airways into the surrounding tissue during inspiration, and little or no liquid reenters during expiration. As a result, FRC accumulates with each breath and the FRC volume increase equals the volume of liquid leaving the airways (36,39) (Figure 9-3). These findings confirm the causal link between spontaneous breathing and FRC accumulation after birth reported using a variety of different animal models (44,52).
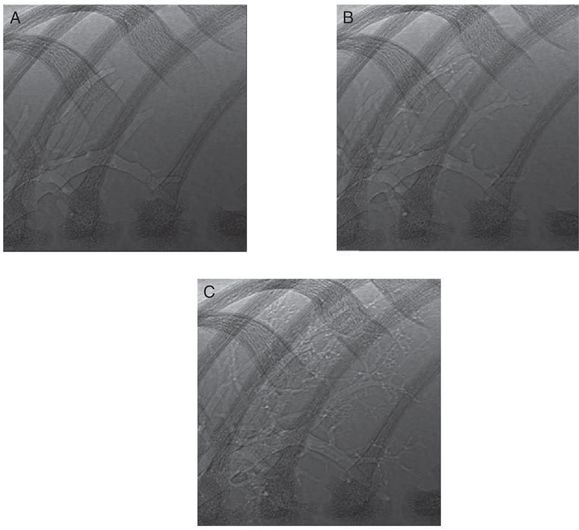
Phase-contrast X-ray images acquired during lung aeration in a spontaneously breathing term rabbit pup. The three images show a close-up view of the non-dependent lower right bronchus for consecutive breaths. (A) Before inspiration, the larger airways are aerated, and the air–liquid interface is visible in the large proximal airways. (B) Following the subsequent inspiration, some smaller airways have aerated, and the air–liquid interface has moved more distally. In between inspirations there is very little or no proximal or distal movement of the air-liquid interface (C).
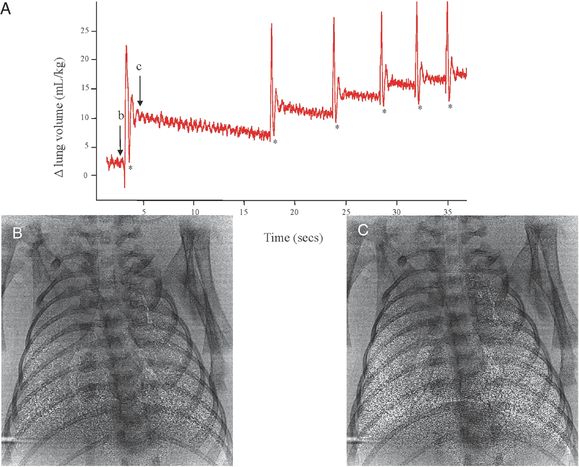
Plethysmograph recording of the first breaths of a spontaneously breathing term rabbit pup after birth. Each rapid large increase in lung volume (in mL/kg) is an inspiratory effort and results in a stepwise accumulation of FRC after each breath. The phase contrast X-ray images were acquired immediately before (image B) and immediately after (image C) an inspiration effort, demonstrating the degree of lung aeration achieved with one breath.
Following the next inspiration, some terminal airways become visible.
Inspiration causes expansion-induced pressure reductions in both the intrapleural space and perialveolar interstitial tissue (36), which generates a pressure gradient between the airways and surrounding tissue (across the airway wall) and between the lower and upper airways. These pressure gradients drive liquid movement distally through the airways and across the epithelium and into the tissue. Within the perialveolar tissue, the liquid forms into perivascular fluid cuffs, from where it is gradually cleared via the pulmonary vasculature and lymphatics (53). As this can take hours, the retention of liquid within the tissue causes pulmonary interstitial tissue pressures to transiently (~4 h) increase (54) (Figure 9-4) and the chest wall to expand immediately after birth (39) (Figure 9-5). Chest wall expansion is required to accommodate the increase in gas volume as well as the volume of liquid within the interstitial tissue that resided within the airways before lung aeration (39). This understanding provides a rational explanation for why an infant needs to have a compliant chest wall at birth. That is, a compliant chest wall will minimize the increase in interstitial tissue pressure associated with increases in intrathoracic volume. If the chest wall was not compliant, the increased pressure required to increase intrathoracic volumes would necessarily increase interstitial tissue pressures to much higher levels and increase the likelihood of liquid reentry into the airways at FRC.
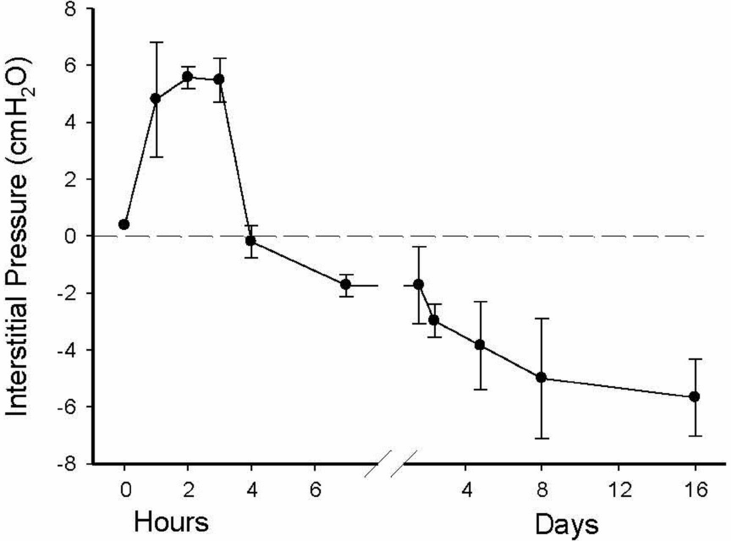
Pulmonary interstitial tissue pressure measured in a term rabbit lung immediately after birth. As the lung aerates, liquid leaves the airways and enters the interstitial tissue compartment at a much greater rate than it is cleared from the tissue by the lymphatics and blood vessels. As a result, pressures initially increase before they decrease and remain subatmospheric.
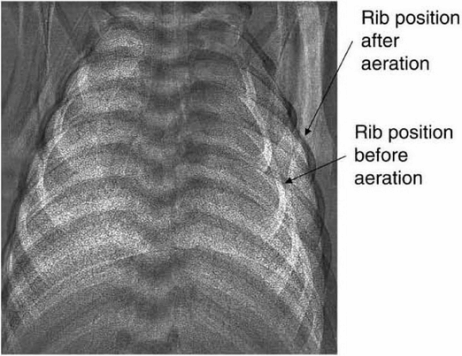
Phase-contrast X-ray imaging showing rib positions before and after lung aeration. The ribs are positioned more horizontally and displaced laterally after lung aeration, indicating a substantially increased thoracic volume caused by the increase in air volume (without the loss of liquid, which has accumulated in interstitium)
Small transient (~4h) increases in interstitial tissue pressure normally occur at birth (54), which facilitates liquid reentry into the airways. This is consistent with a gradual decline in FRC that was noted between breaths (36,50), but the rate of liquid reentry is considerably slower than the rate at which it leaves the airways during inspiration, largely due to the differences in pressure gradients (36, 50). Furthermore, the more airway liquid present at birth, the greater the volume of liquid that must be accommodated in the interstitial tissue compartment. As this compartment has a fixed volume, this will lead to higher interstitial tissue pressures and a greater potential for the reentry of liquid into the airways. This explains why infants born by caesarean section are more likely to have “wet lung” or transient tachypnea of the newborn (44). To prevent reentry of lung liquid these infants perform expiratory braking maneuvers (grunting) and have tachypnea. Continuous positive airway pressure (CPAP) is an effective treatment for “wet lung.”
The Consequences of Lung Recoil
Although lung volumes are commonly thought to be similar after birth and during fetal life, the generation of surface tension when air enters the lung increases lung recoil and causes resting lung volumes to decrease after birth, despite the presence of surfactant. As air is compressible, it is less able to oppose the increase in lung recoil caused by surface tension and, combined with the absence of the distending influence of lung liquid, leads to a reduction in lung expansion (2, 4). This reduction helps to explain a number of physiological changes that occur after birth. For instance, the increase in lung recoil and the partial collapse of the lung away from the chest wall explains why intrapleural pressures become subatmospheric after birth (55). Before birth, intrapleural pressures are similar to ambient (amniotic fluid) pressure (55), but within hours of birth, they decrease to 2–4 cmH2O below atmospheric pressure (54,55). This indicates that the mechanical load experienced by the chest wall has increased with the increase in lung recoil, which likely is important for stiffening the chest wall after birth (56). Similarly, as the liquid is cleared from the interstitial tissue space after birth, interstitial tissue pressures decrease and become subatmospheric and are similar to intrapleural pressures (54). As a result, the interstitial tissue/capillary wall transmural pressures must increase to facilitate capillary recruitment and expansion, which will help sustain the reduction in pulmonary vascular resistance (PVR) after birth (see below).
In addition, the decrease in lung expansion has a profound effect on alveolar epithelial cell (AEC) populations (57). Although increased fetal lung expansion increases the proportions of type-I AECs (57), a reduction in lung expansion leads to increased transdifferentiation of type I AECs into type-II AECs (surfactant-producing cells) (58). The proportion of type-I AECs decrease from 60–65% in the fetus to ~30% in the newborn, whereas the proportion of type-II cells increase from ~30% to 50–55% after birth (59).
Hemodynamic Consequences of Lung Aeration
The fetal circulation has two shunts, the foramen ovale (FO) and ductus arteriosus (DA), which allow both ventricles to work independently and to supply output to the systemic circulation. The FO allows venous return to bypass the right side of the heart and directly enter the left atrium, whereas the DA shunts blood from the main pulmonary artery into the descending aorta. As a result, in fetal sheep, the right ventricle (RV) provides 66% of combined ventricular output, with 33% coming from the left ventricle (LV) (60). Before birth PVR is high and PBF is low (61), and only a small proportion (~10%) of RV output flows through the lungs, with the majority bypassing the lungs and entering the systemic circulation through the DA (right-to-left shunting) (60). Fetal PBF is not persistently low, as is commonly reported, but can vary 10-fold, depending on fetal activity, particularly late in gestation (62). For instance, FBMs significantly increase PBF due to a decrease in PVR, which is thought to result from an increase in the capillary/interstitial tissue transmural pressure (62). This increase in transmural pressure causes capillary distension and recruitment, which increases PBF in close association with inspiratory effort. Each individual FBM causes large changes in the PBF waveform, although the predominant effect is a marked reduction in the amount of retrograde flow during diastole, which is indicative of reduced PVR (62) (Figure 9-6).
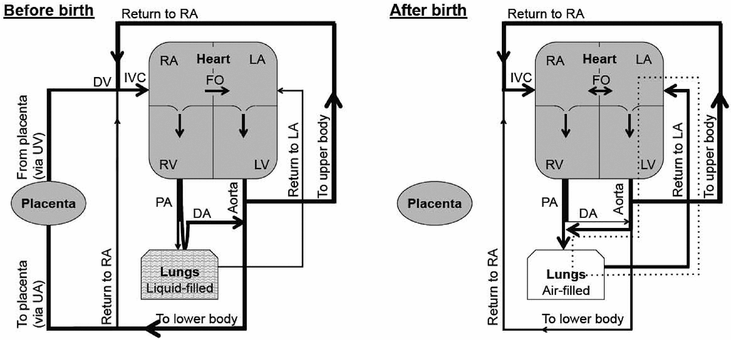
A diagrammatic representation of the circulations before and after birth. Lung aeration after the disconnection of the infant from the placental circulation increases PBF, reverses ductal flow, and establishes a LV-lung-LV short circuit (dotted lines) that maintains LV output. UA; umbilical artery. UV; umbilical vein. DV; ductus venosus. IVC; inferior vena cava. RA; right atrium. LA; left atrium. RV; right ventricle. FO; foramen ovale. LV; left ventricle. PA; pulmonary artery. DA; ductus arteriosus.
The consequence of a high PVR and a low PBF in the fetus is that pulmonary venous return is unable to provide sufficient preload to sustain LV output. Instead, preload for the LV is primarily derived from umbilical venous return in the fetus, which flows via the ductus venous, inferior vena cava, and FO directly into the left atrium (60).
As the umbilical circulation is a low resistance vascular bed, it receives ~30–50% of the combined ventricular output of the fetus (60). As a consequence, umbilical venous return is large (30–50% of total) and supplies the majority of LV preload in the fetus. Thus, clamping the umbilical cord at birth provides a major disturbance to the fetal circulation, particularly to afterloads and the supply of venous return and preload for both ventricles (63). Specifically, removal of the low-resistance umbilical circulation with cord clamping markedly increases downstream peripheral resistance (63). This results in ~30% increase in arterial blood pressure over the first four heartbeats after cord clamping, which in turn causes a transient, pressure-driven increase in cerebral blood flow (63). However, both RV and LV output then markedly decrease (by ~50%), mostly due to the loss of umbilical venous return caused by umbilical cord clamping (63, 64). This large reduction in preload decreases cardiac output and causes a transient decrease in blood pressure (63, 64). Cardiac output remains low, due to the low preload, until ventilation increases PBF. At this time, the rapid and large increase in PBF restores preload to the LV by increasing pulmonary venous return, thereby markedly increasing cardiac output. The increase in PBF also likely restores some preload to the RV, presumably via left-to-right flow through the FO, as RV output also rapidly increases (63).
The increase in PBF at birth is essential for pulmonary gas exchange and to replace umbilical venous return as the primary source of preload for the LV. The increase in systemic vascular resistance with umbilical cord clamping and decrease in PVR with lung aeration has the net effect to decrease PVR below systemic vascular resistance with a reversal in blood flow (from right-to-left to left-to-right) through the DA (64). Within ~10–20 mins of the onset of ventilation in lambs, the extent of the left-to-right shunting through the DA is so large that the LV contributes up to ~50% of PBF, resulting in a substantial LV–lung–LV short circuit within the systemic circulation (64). The consequence of this is an increase in cardiac output, which in turn causes a rebound increase in arterial pressure and carotid blood flow (63). Thus, following umbilical cord occlusion, the loss of umbilical venous return and preload causes substantial reductions in cardiac output (CO), which cannot be restored until the lung aerates and PBF increases. This represents a major disturbance to the fetal circulatory system, resulting in large swings in CO that cause large changes in blood pressures and flows. To avoid these large swings in CO, umbilical cord clamping should be delayed until after ventilation has commenced and PBF has increased. Delayed cord clamping allows the source of preload for the left ventricle to switch from the umbilical circulation to pulmonary venous return without significant interruption (63). As a result, CO does not decrease following cord clamping, and heart rates remain much higher (63). Interestingly, the 30% increase (over four heartbeats) in arterial blood pressure caused by cord clamping is also greatly reduced when cord clamping follows lung aeration, indicating that the decrease in PVR can mostly compensate for the increase in systemic vascular resistance caused by cord clamping (63). This suggestion is consistent with the finding that, following lung aeration, despite a decrease in PVR, shunting through the DA remains right-to-left while the umbilical cord is open (unclamped) (63). This indicates that downstream resistance in the systemic circulation remains lower than the pulmonary circulation. However, immediately (within seconds) after the cord is clamped, flow through the DA switches and becomes left-to-right, indicating that after removal of the umbilical circulation, downstream resistance is lower in the pulmonary circulation than in the systemic circulation (63).
Changes in the Ductus Arteriosus Flow at Birth
After birth, the large decrease in PVR and increase in systemic vascular resistance, reverses the pressure gradient across the DA and blood predominantly shunts from left-to-right (from the systemic to the pulmonary circulation) (64). As a result, retrograde flow in the left and right pulmonary arteries quickly decreases (within minutes of birth), resulting in only forward flow through the pulmonary arteries even during diastole (64). However, the DA flow profile is complex with small amounts of right-to-left shunting persisting during peak systole, whereas for the remainder of the cardiac cycle blood flows mostly left-to-right (64). This transition occurs in ventilated lambs and in spontaneously breathing term infants born by caesarean section (65). While the DA remains open, blood will pass between the pulmonary and systemic circulations, depending on the pressure gradient across this vessel, thereby preventing any substantial decrease in pulmonary arterial pressure. Functional closure of the DA begins within hours of birth in lambs and is clearly evident by the substantial decrease in both absolute DA flow, but most particularly by the pulsatile DA flow caused by ventricular contraction and relaxation (64). Although mediators responsible for DA closure have been identified, it is interesting to speculate whether the anatomic relationship between the pulmonary artery, DA and the descending aorta could be involved. Indeed, considerable turbulence must occur at this site when blood flow through the DA switches from right-to-left to left-to-right, which could elicit the local release of endothelial-derived vasoactive factors that contribute to constriction of the DA.
Strategies to Support Preterm Infants Failing Transition
For the majority of infants, the transition to newborn life is uneventful, and no intervention by the caregiver is needed. However, approximately 3–5% of infants require some form of intervention, usually respiratory support at birth (66). On most occasions resuscitation is needed because respiratory drive is absent (asphyxia) or is insufficient due to immaturity (preterm infants). Adequate ventilation is then the key to successful resuscitation, and chest compressions or medication are rarely needed (67).
Preterm infants (< 32 weeks’ gestational age) have more difficulties aerating their lungs than term infants (38), and approximately 60% of preterm infants need respiratory support at birth (68). Although most preterm infants breathe at birth, hypoxia during labor and at birth can inhibit their respiratory drive. Similarly, inspiratory efforts that are too weak to generate pressures to overcome the high surface tension and frictional forces associated with moving liquid through their airways will not achieve effective lung aeration. In addition, their respiratory muscles are underdeveloped, which contributes to their inability to generate sufficient pressure when there is insufficient surfactant (38). Further, the compliant chest wall leads to inward deformation during diaphragmatic contraction, thereby reducing the inspired tidal volume. The chest wall is also unable to resist lung recoil, which reduces resting lung gas volumes at end expiration (69, 70). Also adrenaline driven ENaC activation, which assists in preventing lung liquid returning to the airways, is largely absent in preterm infants, who are less able than term infants to maintain airways free of liquid (71,72).
Facilitating Lung Liquid Clearance and Lung Aeration in Preterm Infants
As the resistance to liquid flow through an airway is about 100 times greater than air, for a given pressure gradient the flow of liquid will be orders of magnitude slower than air. To overcome this higher resistance, either higher pressures or longer inflation times (i.e., greater pressure-time integral) are needed. To avoid the use of high pressures, a theoretically better alternative is to prolong the time over which the inflation pressure is applied (sustained inflation) (73). Our natural tendency is to think that inspiration should be brief and followed by expiration, as the function of expiration is to clear the lungs of CO2. However, when the distal airways are liquid filled, no gas exchange can occur, and so expiration serves no purpose.
In a series of preterm animal studies, sustained inflation pressures for up to 20 s duration aerated the lungs from the first inflation. Sustained inflations were also associated with more uniform aeration across the lung, a larger FRC and more consistent volume during consecutive tidal ventilation (74–76). Similarly, sustained inflations of 5 s were effective at aerating the lung of intubated asphyxiated term infants (77). Currently only limited clinical data on the effects of sustained inflations for ventilating preterm infants at birth are available. Lindner reported in a retrospective cohort study the use of a sustained inflation of 15–20 s in a noninvasive approach in very preterm infants (78). The study was repeated in a small randomized trial comparing a sustained inflation of 15 s with standard PPV. Although recruitment was slow and the study was stopped early, there was a trend for a lower rate of subsequent CPAP-failure in the sustained inflation group (79). Harling et al. randomly assigned preterm infants (n = 52) to a 2 versus a 5 s initial sustained inflation and found no difference in pro-inflammatory pulmonary cytokines as the primary outcome (80). Te Pas et al. used a sustained inflation of 10 s followed by CPAP in a delivery room strategy with a nasal tube and neopuff as compared to standard mask and bag ventilation. The rate of infants needing intubation and mechanical ventilation and the rate of bronchopulmonary dysplasia (BPD) decreased, but the specific contribution of sustained inflation was not isolated in this latter study (81). More recently a strategy using initial sustained inflations with positive end expiratory pressure (PEEP)/CPAP immediately after birth reduced the rate of intubation/mechanical ventilation and BPD in preterm infants as compared to historical controls using intermittent positive pressure ventilation (IPPV)/CPAP (82).
No specific recommendations have been made for the use of sustained inflations in international resuscitation guidelines. There are concerns of overinflation, which can cause lung injury in animals when using high inflation pressures (83, 84). However, sustained inflation did not cause overdistention in the imaging studies that accurately monitored lung distension (74, 75). Similarly the concern that a sustained inflation will impede the increase in PBF at birth was not confirmed in a preterm lamb study. The increase of PBF at birth was similar in lambs given a sustained inflation (40 cmH2O for 1 min or to a volume of 20 mL/kg) or ventilated with conventional PPV (76). However, oxygenation and respiratory function were markedly improved in the sustained inflation group. Similarly, no relevant adverse effects using sustained inflations, such as increased rates of pulmonary air leaks, intraventricular hemorrhage (IVH) or negative hemodynamic effects were reported in the randomized trials (79, 81, 82). On the contrary, Fuchs et al. demonstrated with near-infrared spectroscopy (NIRS) measurements that sustained inflation in preterm infants at birth increased cerebral oxygen saturation at least as fast as in full-term infants not requiring any respiratory support (85). In preterm lambs a sustained inflation is just as injurious as conventional PPV, although the peak inflating pressures used in that study were 50 cmH2O, which may have resulted in some overdistension (86). Another recent preterm lamb study indicated that a prolonged (over minutes) lung recruitment strategy that used stepwise increases (to 20 cmH2O) and decreases in PEEP was a more effective strategy than a sustained inflation for improving respiratory function at birth (87). However, considering that this strategy will be applied for minutes and that high PEEP levels can have severe effects on PBF (see following), this approach is likely to have a major detrimental impact on cardiovascular function. Further studies are needed to assess the appropriate pressure and inflation duration that will effectively aerate the lung without causing injury.
Maintaining FRC after Birth
To maintain FRC immediately after birth, preterm infants have to oppose two major counterforces: the increase in lung recoil caused by surface tension and supra-atmospheric pressures within the interstitial tissue that promote liquid reentry into the airways. Preterm infants frequently use expiratory braking maneuvers to prevent distal airway collapse and/or to prevent liquid from entering the airways (37,50). The infant actively expires against a closed/adducted glottis, thereby sustaining a supra-atmospheric pressure in the airways to prevent loss of FRC. In addition, by reducing surface tension within the surface film lining the internal alveolar surface, surfactant makes the pressure within this film less negative and thereby reduces the transepithelial pressure gradient for alveolar flooding. When the respiratory effort and surfactant are insufficient, an external end expiratory pressure can be applied via mask or tube as a CPAP or PEEP during ventilation (Figure 9-7) (73) There are very little data on the optimal PEEP/CPAP level at birth, but undoubtedly the pressure will vary between infants and with the time after birth. Indeed, the optimum PEEP/CPAP level will be a counterbalance between respiratory and cardiovascular effects. For instance, in preterm lambs ventilated from birth, PEEP levels above 8 cmH2O improve oxygenation but adversely affect PBF by increasing PVR. As a result the PBF waveform is markedly altered, reestablishing retrograde flow during diastole, which is characteristic of the fetal state (88). Recent phase-contrast X-ray imaging studies have shown that a more uniform distribution of ventilation can be achieved by initiating ventilation with a high PEEP level of 10 cmH2O (89). After lung aeration, small and stepped reductions in PEEP result in more uniform changes in ventilation than do starting with lower PEEP levels and then increasing the PEEP (89). To avoid mechanical ventilation–associated injury, many caregivers prefer the gentle approach of using noninvasive ventilation. Several large randomized trials demonstrated that early CPAP after birth is a good alternative for endotracheal intubation, mechanical ventilation, and surfactant treatment. However, in the trials this “early” CPAP is applied after most of the infants were stabilized with PPV (90).
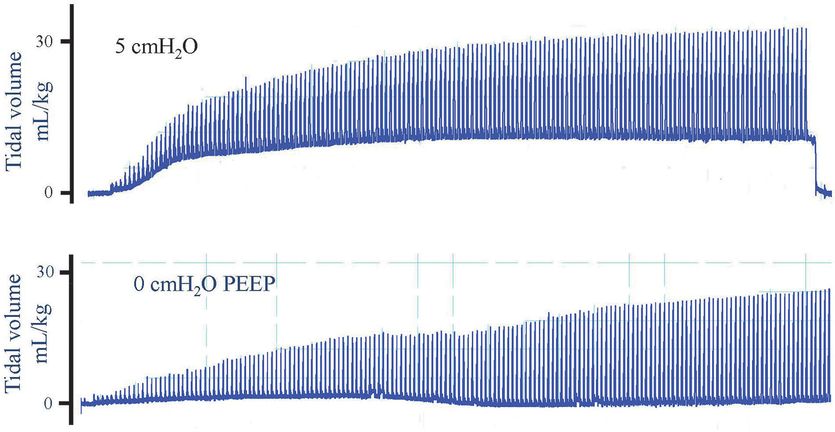
Plethysmography recordings of preterm rabbit pups ventilated from birth with (top panel) or without (bottom panel) PEEP. When ventilated with 5 cmH2O, PEEP lung volume at end expiration increases after which it remains stable. In contrast, when a pup was ventilated without PEEP (0 cmH2O), no FRC accumulated. The estimated dead space volume is indicated by the dotted line, indicating that volume in the lung only increased above the dead space volume briefly during inspiration with 0 PEEP. As result, gas exchange is restricted to the brief period during inflation and does not occur throughout the respiratory cycle.
The Use of Oxygen
Hyperoxemia (high oxygen levels in blood) can lead to hyperoxia (high oxygen concentrations in tissue), causing oxidative stress and tissue injury (91,92). Excessive oxygen exposure should be avoided in infants during stabilization at birth. Meta-analyses indicate that resuscitation of term infants at birth with air significantly reduced mortality compared with infants resuscitated with 100% oxygen (91–96). Recently updated international resuscitation guidelines now recommend that respiratory support in term infants should start with air (92,97,98). Less clinical data are available for preterm infants, although hyperoxia at birth may increase the risk of BPD (99). As a result, current recommendations suggest using oxygen judiciously during stabilization of preterm infants at birth (92,97,98). On the other hand, hypoxia is known to inhibit breathing in the fetus (16) due to a direct inhibitory input into the respiratory center from higher brain centers (16). Although a temporal change in O2 sensitivity occurs in days/weeks after birth (5) and most preterm infants breathe at birth (34,100), it is not known when the switch from respiratory suppression to stimulation occurs in response to hypoxia. It is possible that hypoxia immediately after birth will cause a weakened or absent respiratory drive, particularly in preterm infants, who are essentially exteriorized fetuses. Indeed, maturation of the hypoxic sensitivity for breathing is delayed in preterm lambs (5).
The optimal inspired oxygen content required to avoid hypoxia as well as hyperoxia remains unclear. In small randomized trials, hyperoxia occurred more often in preterm infants when resuscitation started with high oxygen concentrations (99, 101–103). However, most infants started with 21% or 30% oxygen required increases in inspired oxygen levels to meet the target oxygen saturation (SpO2) in the first minutes of life (99,101–103). However, these studies were performed before the normograms of SpO2 percentiles (104) were introduced and higher target ranges were used (99, 101–103).
It is commonly assumed that the sensitivity of the pulmonary vasculature to oxygen differs between term and preterm infants and persistent hypoxia is caused by a failure of the pulmonary vasculature to sufficiently dilate at birth in the absence of supplemental oxygen (103). However, experimental studies have shown that the decrease in PVR at birth is mostly related to ventilation onset and that oxygen has a much smaller impact, which appeared to be additive with ventilation (76,105,106). Also, Sobotka et al. found that increasing FiO2 to 1.0 in lambs who were hypoxic and difficult to ventilate markedly improved blood oxygenation, but had no effect on ventilation variables (lung compliance), PaCO2, or PBF (76). This supports the hypothesis that increased oxygenation after increasing the FiO2 to 1.0 is achieved by increasing the partial pressure gradient for oxygen diffusion across the air/blood barrier to compensate for a limited surface area and associated ventilation perfusion mismatch (76).
Mask Ventilation
The success of ventilation strategies to support respiratory function at birth in the newborn is largely dependent on the technique and strategy applied. In most neonatal units, the initial approach is to use noninvasive ventilation that is applied using a face mask. In studies using respiratory function measurements, face mask ventilation was difficult, and the delivered tidal volumes were often inadequate (34,107). Mask leak and obstruction frequently occur and together with the use of inadequate pressures, the tidal volumes are often below dead space volume (34,107). Furthermore, although experimental studies have demonstrated beneficial effects of applying an initial sustained inflation at birth, it has been difficult to duplicate these benefits with mask ventilation in preterm infants at birth due to similar problems (108). Although the source of the obstruction is unclear, it is possible that during the pressure inflation the infant’s glottis is closed. As a result, when the infants take a breath during the sustained inflation, the glottis opens, and large volumes enter the lung. In some apneic infants, breaths appeared to be triggered during the sustained inflation but then the infant remained apneic. It is possible that applying a pressure to the pharynx during the sustained inflation may have induced a respiratory reflex that stimulated breathing, as demonstrated in cats (109,110), and that this stimulus was removed following the sustained inflation (Figure 9-8). It is also possible that the pressures used for the sustained inflations were too low to overcome the resistance of the liquid-filled lung. Before we can translate the beneficial effects of a sustained inflation into clinical practice, we need to develop more effective strategies that will allow the inflation pressures developed during a sustained inflation to reach the airways.
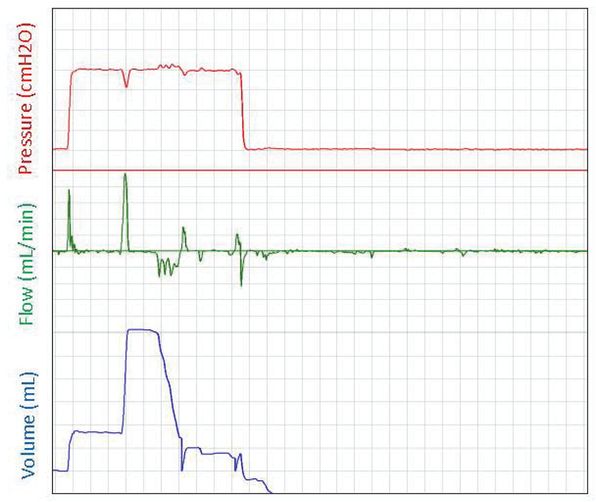
A respiratory function recording of an initial sustained inflation given to an apneic preterm infant at birth. The pressure is displayed in the top panel (cmH2O), gas flow into and out of the face mask is displayed in the middle panel (mL/min), and the gas volume (mL) moving into the lung is displayed in the bottom panel. Large breaths are observed when the sustained pressure is applied, but the infant remains apneic after the sustained inflation.
Considering the difficulties caregivers have with the use of face masks, alternative interfaces have been suggested, including a nasal tube (111,112). The recently completed MOUNTAIN trial compared the effect of face masks versus nasal tubes during noninvasive ventilation at birth (113). As the rates of intubation were similar between the groups and complications of using each interface were infrequent, the trial concluded that either interface could be used and that a nasal tube may be a good alternative to a face mask (113). However, measurements of respiratory function during nasal tube ventilation showed that the tidal volumes were often too low. The low tidal volumes resulted from high rates of leak and obstruction as compared with face mask ventilation. Leak-free ventilation was more difficult with a nasal tube, even when the contralateral nostril and mouth were closed. It is also possible that the tip of the tube was against the posterior wall of the nasopharynx, causing obstruction.
Recent studies have demonstrated that during resuscitation at birth the mask ventilation given was not effective until the infant took a breath (34, 107). However, breathing is difficult to observe and is therefore often missed clinically, especially in preterm infants covered in a wrap to prevent hypothermia (114). Respiratory function monitoring (RFM) can be used to detect spontaneous breathing (34,114,115), and although the spontaneous breaths are not always at a sufficient rate or tidal volume for detection, spontaneous breaths usually yield larger tidal volumes than mechanical inflations. In addition, when a spontaneous breath coincides with the mechanical inflation, the resulting tidal volume is much larger. Although the presence of breathing in preterm infants likely influences the caregiver’s decision for whether additional ventilation is required, caution is needed when giving these inflations. In particular, when the breaths and inflations occur in and out of synchrony in a random manner, problems can arise. When inflations and spontaneous breaths coincide, inadvertently high transpulmonary pressures can occur, resulting in high tidal volumes and an increased risk for lung injury and air leaks (34,107,116). On the other hand, when they do not coincide, the inflation pressure may be too low to produce a sufficient tidal volume. The caregiver then may increase the inflation pressure, which may be injurious when the spontaneous breath occurs with the mechanical breath (117).
Ventilation Induced Injury at Birth
Although most clinical trials in delivery room management have failed to show a decrease in morbidity and mortality, there is much experimental data demonstrating that the respiratory support given at birth can injure the preterm infant, with potentially lifelong consequences. In addition, large clinical studies in very preterm infants reported higher mortality rates and an increased risk of lung and brain injury with increasing levels of delivery room resuscitation (118–120). At birth, the lungs of very preterm infants are uniquely susceptible to injury because they are structurally immature, surfactant deficient, liquid filled, and not supported by a stiff chest wall (38). The gas volume and compliance of the lung in newborn infants greatly changes over the first few breaths as airway liquid is replaced with air. This highlights the large differences in regional lung mechanics that occur when the lung only partially aerates (121). Spontaneously breathing infants develop high transthoracic pressures over the first few breaths, and relatively high positive pressures are required to initiate mechanical ventilation (6). However, compliance rapidly increases as more of the lung aerates, resulting in lower pressure requirements to achieve a functional tidal volume (VT) with subsequent breaths. In addition, although the lung, heart, and brain are often considered independently, they are intimately linked, particularly during transition at birth. Treatments aimed to provide respiratory support for the lungs can have severe adverse consequences for the preterm heart and brain (122). For example, PPV not only can cause lung injury, but also can adversely affect the cardiovascular system, systemic circulation, and cerebral circulation. Similarly, inflammation resulting from lung injury can induce a systemic inflammatory response that includes the brain (122).
Lung Injury During Clearance of Lung Liquid and Lung Aeration
At birth, the liquid-filled immature lungs of preterm infants are very vulnerable to injury. When tidal ventilation is applied to a partially liquid-filled lung, the inflation pressure and the short inflation time (usually < 0.5 s) may be sufficient to only inflate aerated lung regions. In liquid-filled regions, the pressure-time integral of normal ventilation is far too low to overcome the resistance to moving liquid distally through the airways. Instead, gas only flows into aerated regions, overdistending them and causing regional volutrauma. For example, 5 mL/kg equates to 15 mL/kg if only one-third of the lung is aerated and the remaining two-thirds are liquid filled. Combined with a compliant chest wall, regional overdistension injury at a given airway pressure may be large.
The site of injury in the lung is not restricted to the distal gas exchange regions, as smaller conducting airways that contain little collagen are also susceptible to injury (123,124). In preterm sheep, airway stretch occurs during initiation of ventilation, and initial injury is localized primarily to the bronchioles and respiratory bronchioles (124). Also, the shear stress caused by the movement of the air/liquid interface across the epithelial cells can distort and injure the epithelium of the small airways. As the initial ventilation may be given before much of the endogenous surfactant is secreted, there may be no protective effect of surfactant (125).
Preterm infants have a smaller inspiratory reserve volume than term infants, and therefore the volume difference between FRC and total lung capacity (TLC) is smaller (126). Preterm infants have an FRC of 11 mL/kg and a TLC of 19 mL/kg (13), while term infants have an FRC of ~20 mL/kg and a TLC of 43–52 mL/kg (126,127). Thus, in preterm infants, a tidal volume above 8 mL/kg may distend the lung above TCL and cause injury (123,128). This is because spontaneously breathing preterm infants have a mean tidal volume of 4.4 (range 2.6–7.2) mL/kg (37). As five large rapid inflations at birth can cause lung injury (83) and abolish the benefits conferred with surfactant treatment (125), it is often assumed that overinflation per se is the root cause of ventilation-induced lung injury. Indeed, injurious ventilation, using large tidal volumes induces acute phase injury response genes (129). However, the tidal volumes used in those studies were very large, either 15 mL/kg (129) or 35–40 mL/kg (83), and therefore, it is not surprising that they caused injury. As high inflation rates, causing large shear stress, are also injurious (130), it is not known whether it is large tidal volumes per se or the high rate at which these volumes are achieved that is primarily responsible for the injury.
Currently caregivers use pressure-limited devices for resuscitation in the delivery room, but the tidal volume is not measured. Instead of measuring volume, caregivers rely on chest excursions, which are not a good indicator of tidal volume (128). Many infants can receive inappropriate VT within minutes of birth (117). Indeed, Dawson et al. measured the VT delivered during resuscitation of preterm infants at birth with a T-piece or self-inflating device. The tidal volume ranged from 0 to >30 mL/kg (131), with the majority (85%) of preterm infants receiving excessively high VT (> 8 mL/kg) (131).
It will be difficult to define the safe tidal volume range during mask ventilation of preterm infants at birth for two main reasons. First, the current recommendation of the safe range of tidal volumes (4–8 mL/kg) is based on measurements of spontaneous breathing and intubated and ventilated infants (132). However, during mask ventilation, the complete respiratory system is pressurized and ventilated, which includes the lungs, the trachea, and the nasopharynx. Some gas also may enter the esophagus. During an inflation given via a mask or nasal tube, the nasopharynx is pressurized, leading to a volume displacement, which does not occur during a spontaneous breath (132). As such this volume displacement has to be taken into account when measuring volumes during mask ventilation (132). Mask ventilation may be more effective if the caregiver aims for larger tidal volumes than would be targeted in intubated infants. Thus, a different range of safe tidal volumes should be defined for ventilation given via a mask or an ET tube to avoid inadequate but also excessive and injurious tidal volumes (132).
Lung Injury Following Lung Aeration
Repeated collapse and reopening of distal airways will expose the lung to high shear forces leading to injury (atelectrauma) (133), which initiates an inflammatory cascade that includes cytokine release and the recruitment of activated leukocytes to the lungs (133). The damage is characterized by epithelial disruption, hyaline membrane formation, airway cell loss, increased alveolar capillary permeability, surfactant dysfunction, decreased lung compliance, and poor gas exchange (134). This sequence emphasizes the importance of applying an adequate PEEP/CPAP level at birth to prevent airway collapse. In surfactant-treated preterm lambs, PPV without PEEP was associated with reduced compliance and oxygenation compared with ventilation with PEEP of 4 to 7 cmH2O (135). In addition, preterm lambs ventilated with a PEEP of 8 cmH2O had a significantly lower oxygen requirement by 10 minutes of age compared with lambs ventilated with no PEEP (136), and the improvement in oxygenation occurred more rapidly than with surfactant administration (137).
Hemodynamic Consequences
Ventilation can alter pulmonary venous return and, as a result, cause rapid changes in left ventricular output and systemic arterial pressure. As instantaneous cerebral blood flow is pressure passive in the immature brain at birth, large, rapid fluctuations in cardiac output cause large swings in cerebral blood flow (63). Experimental and clinical studies showed the correlation between mean airway pressure and altered PBF leading to destabilization of systemic arterial flows (138–141). In addition, high airway pressures can also compress the heart directly and reduce cardiac performance and ventricular output (141, 142), Changing the PEEP level can significantly change the systemic blood flow in preterm infants (143). Echo Doppler studies in the first 24 h after birth in preterm infants (< 29 weeks) demonstrated a strong association of low SVC flow with cerebral injury, including IVH and long-term neurodevelopmental disability (144,145).
In preterm lambs, large fluctuations in cerebral blood flow occurred when high VT ventilation was given for the first 15 minutes after birth (122), which was accompanied by impaired cerebral autoregulation (cerebral vasoparalysis) and increased vascular extravasation (leakage), a precursor to cerebral hemorrhage (122). Optimizing the initial respiratory support at birth (immediate surfactant, initial sustained inflation followed by tidal ventilation of 7 mL/kg) significantly reduced the risk of brain injury by improving CBF stability and cerebral oxygenation and reducing vascular permeability (76,122). It is not ventilation per se that is injurious, but the poorly controlled use of PPV in the delivery room that increases the risk of brain injury.
Systemic Consequences of Lung Injury
Initial ventilation in preterm lambs with higher than normal tidal volumes causes pulmonary inflammation (146) and subsequently a systemic inflammatory cascade (123,147,148). Indeed, increased levels of pro-inflammatory cytokines IL-8, IL-1, and TNF and decreased anti-inflammatory cytokine IL-10 were measured in preterm and term newborns 2 h after initiation of mechanical ventilation (149). The circulating cytokines elicited and exacerbated an inflammatory response within the brain (150,151), which resulted in increased infiltrating inflammatory cells, activation of resident microglia, increased oxidative stress, and subsequent diffuse white matter gliosis within the periventricular white matter, subcortical white matter, and corpus callosum (122,152). The pattern of white matter injury associated with increased systemic pro-inflammatory mediators is consistent with that in infants with PVL associated with high plasma or cerebrospinal fluid cytokine levels (153,154). Increased systemic pro-inflammatory cytokines not only cause direct cerebral injury but may also alter postnatal hemodynamics as cord-blood IL-6 levels are inversely related to systolic, mean, and diastolic blood pressures (155). Also the gray matter can be injured with high tidal ventilation, as demonstrated in animal experiments (148,156,157), and the injury was reduced with less-invasive ventilation (156,157).
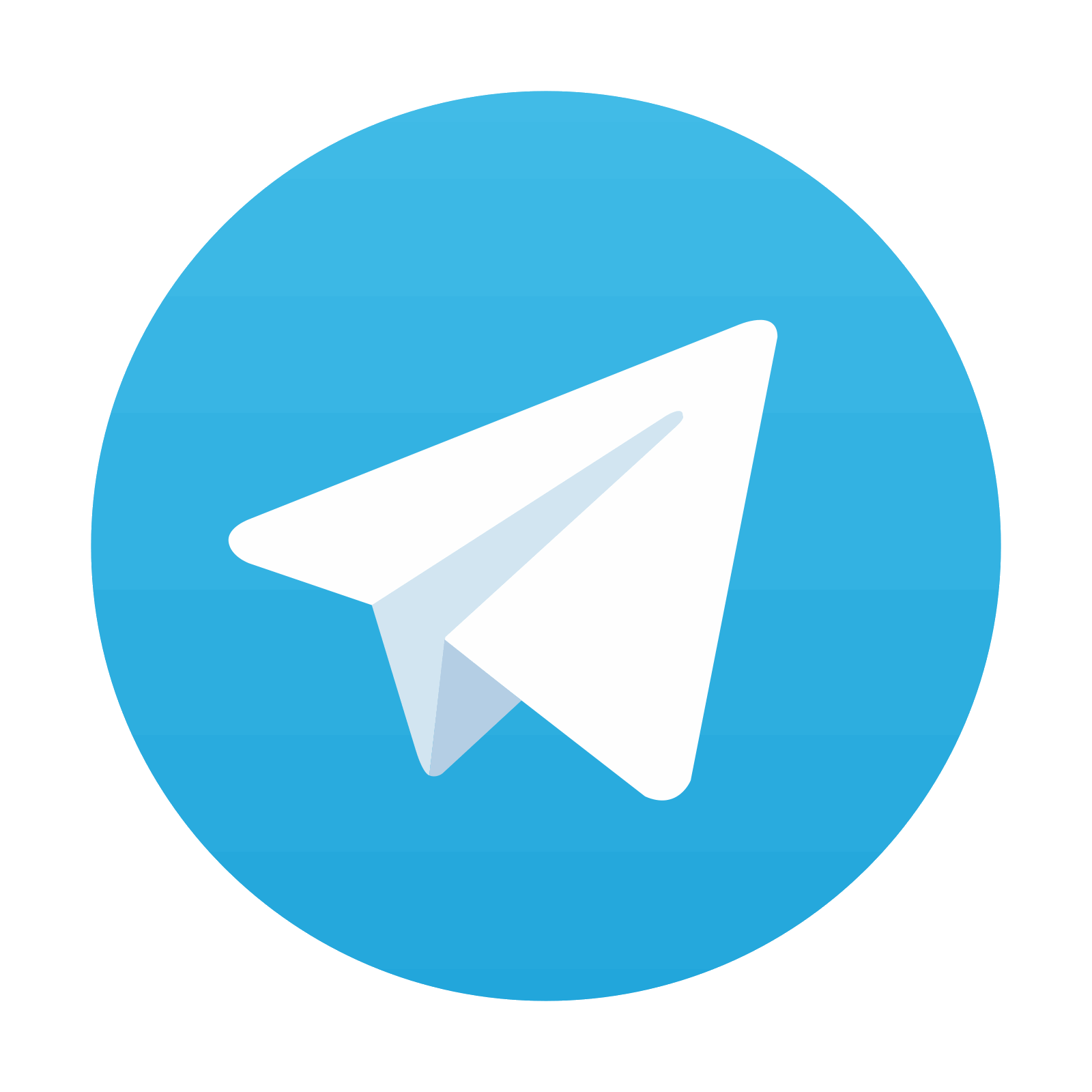
Stay updated, free articles. Join our Telegram channel
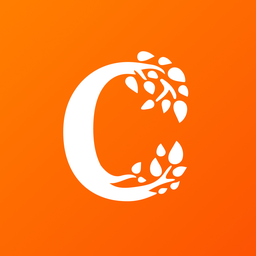
Full access? Get Clinical Tree
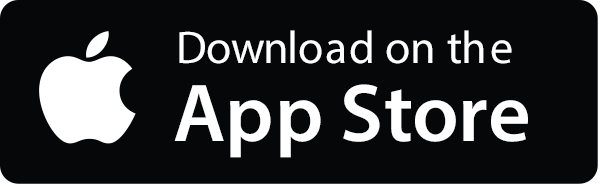
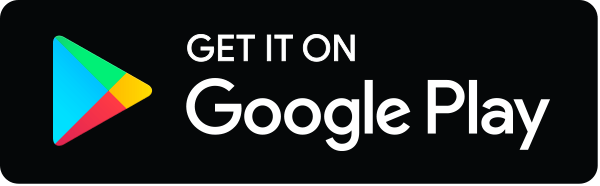