Hypoplastic Left Heart Syndrome
James S. Tweddell
George M. Hoffman
Nancy S. Ghanayem
Michele A. Frommelt
Kathleen A. Mussatto
Stuart Berger
Epidemiology and Etiology
Hypoplastic left heart syndrome (HLHS) makes up 1.4% to 3.8% of congenital heart disease (CHD), an incidence of 0.016% to 0.036% of live births. Despite this low incidence, HLHS causes 23% of cardiac deaths during the first week of life and 15% of cardiac deaths within the first month of life (1,2,3,4,5,6). A male predominance has been reported for HLHS (55% to 67%) (1,2,7,8,9,10).
While no gene abnormality is specific to HLHS there is strong evidence supporting a genetic etiology for HLHS. The recurrence risk in families with one affected child is 0.5% to 2%. Additionally, the recurrence risk for other forms of CHD in families with one affected child with HLHS is 2.2% to 13.5% (11,12,13). Pedigree analyses have demonstrated a 12% prevalence of cardiac abnormalities involving the left ventricular outflow tract in first-degree relatives of patients with HLHS (14,15). Lewin et al. (16) studied 278 first-degree relatives of 113 patients with nonsyndromic left ventricular outflow obstruction and found that 4.6% had a bicuspid aortic valve; an additional 11.5% of relatives had anomalies of the aorta, aortic valve, left ventricle, or mitral valve.
Extracardiac anomalies and genetic syndromes have been recognized in patients with HLHS with a reported incidence of 15% to 30% (17,18,19,20). Identified heritable syndromes associated with HLHS include, Kabuki syndrome, Noonan syndrome, Smith–Lemli–Opitz syndrome, Holt–Oram syndrome, Ellis–van Creveld syndrome, oral–digital–facial syndrome CHARGE syndrome and PAGOD syndrome (1,21,22,23,24,25,26,27,28,29). Trisomies and monosomies associated with HLHS include; Turner syndrome, trisomy 13, trisomy 18, and trisomy 21. Other chromosomal abnormalities associated with HLHS include; duplication of short arm of chromosome 12, 2q-, a balanced 3:7 translocation, 4q-, 4p-, 7q-, 11q- (Jacobsen syndrome), duplication of 16q, 16p12.1 microdeletion, 18p-, 21q22.3 deletion (30) and 22q11.21 microduplication (1,26,31,32). Specific gene mutations implicated in HLHS include; GJA1 (Connexin Protein 43), NKX2–5, NOTCH1, FOXP1, and MCTP2 (33,34,35,36,37,38). Linkage analyses have suggested additional loci on chromosomes; 2p23, 10q21, 16p12, 2p15, 10q22 and 6q23 (39,40,41). More recently gene copy number variants have been implicated including; GATA4, MYH11, GJA5, SMC1A, MFAP4, and CTHRC1 (42,43,44,45). Future work in molecular genetics may allow determination of the cause of HLHS and will be assisted by population registry information tissue banking and improved genomic sequencing technologies.
Environmental factors have been implicated in the development of HLHS. The Baltimore-Washington Study identified a geographic cluster of HLHS in a region of Baltimore characterized by industrial land use and release of solvents, polychlorinated biphenyls, and dioxin into air (46). Environmental factors were also implicated in a study finding an increased incidence of HLHS in southeastern Wisconsin (47). The rate of HLHS births is higher in rural and urban areas compared with suburban areas (48). Some studies have identified a seasonal variation with higher rate of HLHS births in spring and summer (48,49). The seasonal variation suggests that an infection may play a role in the development of HLHS. A link between maternal group A beta-hemolytic streptococcal pharyngeal infection and HLHS has been suggested (50). Antibodies formed from Streptococcal pharyngitis cross the placenta and are associated with injury to the developing left ventricular outflow tract of the fetus resulting in HLHS. A recent study found a seasonal occurrence to HLHS again implicating an infectious-environmental cause (51).
The Developing Fetus: Echocardiography and Intervention
Prior to the advent of fetal echocardiography, the embryologic cause of HLHS was not entirely clear. However, with advances in fetal cardiac imaging, it became evident that many forms of CHD evolve throughout gestation. In 1989, Allan (52) observed the in utero evolution of HLHS in a fetus initially diagnosed with critical aortic stenosis. A similar case report was published by Danford (53) in 1992. Since that time, several fetal cardiac centers have reported retrospective collaborative data that suggest that serial measurements of left heart growth and assessment of flow direction across the foramen ovale and distal aortic arch may identify fetuses at risk for severe left heart hypoplasia at term (53,54,55,56). It is now postulated that many cases of HLHS are dynamic and progressive throughout gestation, resulting from altered left ventricular outflow (aortic stenosis) or altered left ventricular inflow (mitral valve stenosis/foramen ovale restriction/alterations of atrial septal anatomy) (57,58,59). The field of prenatal cardiac intervention is just beginning, but early recent successes with balloon dilation of the aortic valve suggest that the development and incidence of HLHS at term can be altered (60).
Fetal Echocardiography
Recent advances in two-dimensional and Doppler echocardiography have made it feasible to diagnose all forms of CHD in the fetus. HLHS is one of the most common structural lesions diagnosed prenatally, as a screening obstetric ultrasound will preferentially identify lesions that dramatically alter the four-chamber view (61,62,63) (Fig. 46.1). The prenatal diagnosis of HLHS is easily made when a small, muscle-bound left ventricular chamber is identified. The challenge for the fetal echocardiographer today is to recognize the potential for the evolution of HLHS, especially since some of these patients may be candidates for prenatal intervention. Another challenge is to diagnose the severely restrictive or intact atrial septum in this patient group prior to birth, as these patients have a particularly dismal outcome and may also benefit from prenatal intervention.
The fetal left ventricle is predominantly filled with oxygenated blood that returns from the placenta and traverses the foramen ovale (64). If blood flow across the foramen ovale is diminished or reversed, the combined cardiac output is redistributed to the right ventricle and pulmonary artery, resulting in enlargement of the right heart structures and creating less impetus for normal growth of left heart structures, possibly evolving into HLHS. Perhaps the well-recognized mechanism for decreased flow or reversal of flow through the foramen ovale in utero is the presence of severe aortic valve disease (52,53,54,55,56). With significant aortic valve stenosis,
alterations in left ventricular compliance may occur, either secondary to the development of left ventricular hypertrophy or secondary to the development of left ventricular dilation and dysfunction. Endocardial fibroelastosis (EFE), a poorly understood phenomenon where the endocardial lining of the left ventricle becomes fibrotic, may also be present. As the disease state progresses, with subsequent elevation in left atrial pressure, flow across the foramen ovale becomes bidirectional and eventually left to right, the result of which may be the cessation of left ventricular growth (65). In a classic study by Hornberger et al. (55), the prenatal and postnatal echocardiograms of 21 fetuses with left heart obstructive lesions were reviewed to identify possible prenatal indicators of postnatal disease severity. Prenatal indices that correlated with HLHS at birth included a smaller mitral valve and ascending aorta in the midtrimester, as well as a decreased rate of growth for all left heart structures. Other prenatal features included reversal of flow across the foramen ovale and retrograde ductal supply of the distal aortic arch. In a more recent study, Makikallio et al. (66) reviewed the natural history of aortic stenosis in 43 fetuses initially referred prior to 30 weeks gestation. At the time of the initial examination, the LV:RV length ratio was >0.8:1, and aortic stenosis was the dominant lesion. The presence of moderate left ventricular dysfunction, retrograde transverse aortic arch flow, left-to-right atrial-level shunting, and a monophasic mitral inflow on the initial prenatal echocardiogram were found to be risk factors for the development of HLHS. Again, in this study there was decreased rate of growth of all left heart structures in those patients who developed HLHS. Based on these two series, it is now clear that the fetus with aortic stenosis is at risk for the development of HLHS. Serial echocardiographic follow-up is indicated in these fetuses, paying particular attention to growth of left heart structures and patterns of blood flow across the foramen ovale and transverse aortic arch. Importantly, it now seems feasible to reliably select fetuses for prenatal intervention, using both anatomic and physiologic markers.
alterations in left ventricular compliance may occur, either secondary to the development of left ventricular hypertrophy or secondary to the development of left ventricular dilation and dysfunction. Endocardial fibroelastosis (EFE), a poorly understood phenomenon where the endocardial lining of the left ventricle becomes fibrotic, may also be present. As the disease state progresses, with subsequent elevation in left atrial pressure, flow across the foramen ovale becomes bidirectional and eventually left to right, the result of which may be the cessation of left ventricular growth (65). In a classic study by Hornberger et al. (55), the prenatal and postnatal echocardiograms of 21 fetuses with left heart obstructive lesions were reviewed to identify possible prenatal indicators of postnatal disease severity. Prenatal indices that correlated with HLHS at birth included a smaller mitral valve and ascending aorta in the midtrimester, as well as a decreased rate of growth for all left heart structures. Other prenatal features included reversal of flow across the foramen ovale and retrograde ductal supply of the distal aortic arch. In a more recent study, Makikallio et al. (66) reviewed the natural history of aortic stenosis in 43 fetuses initially referred prior to 30 weeks gestation. At the time of the initial examination, the LV:RV length ratio was >0.8:1, and aortic stenosis was the dominant lesion. The presence of moderate left ventricular dysfunction, retrograde transverse aortic arch flow, left-to-right atrial-level shunting, and a monophasic mitral inflow on the initial prenatal echocardiogram were found to be risk factors for the development of HLHS. Again, in this study there was decreased rate of growth of all left heart structures in those patients who developed HLHS. Based on these two series, it is now clear that the fetus with aortic stenosis is at risk for the development of HLHS. Serial echocardiographic follow-up is indicated in these fetuses, paying particular attention to growth of left heart structures and patterns of blood flow across the foramen ovale and transverse aortic arch. Importantly, it now seems feasible to reliably select fetuses for prenatal intervention, using both anatomic and physiologic markers.
Although the operative survival for infants born with HLHS has improved significantly over time, the subgroup of patients with a highly restrictive or intact atrial septum continues to experience a higher mortality (67,68). These infants can be profoundly cyanotic at the time of delivery and are often unresponsive to medical intervention. Even with prompt resuscitation and adequate decompression of the atrial septum, there is ongoing morbidity and mortality, likely related to secondary anatomic changes in the lung. Some investigators have reported “arterialization” of the pulmonary veins and lymphatic dilation in this setting; others have postulated that there is associated pulmonary artery hypoplasia. The ability to diagnose a restrictive atrial septal defect prior to birth would allow more accurate prenatal counseling and planning immediate postnatal intervention. Theoretically, prenatal catheter intervention in this subgroup of patients may alter the secondary anatomic changes in the lung, possibly improving long-term outcome. For all of these reasons, routine evaluation of the atrial septum should be performed in all fetuses with HLHS. Direct assessment of foramen ovale size has not correlated well with the degree of left atrial hypertension at the time of birth, likely a reflection of the inability to clearly visualize the defect, which often lies more superiorly and posteriorly in the left atrium (69). Doppler interrogation of the pulmonary veins is technically much simpler, and the pattern of pulmonary venous flow in HLHS has correlated well with left atrial hemodynamics (69,70). The normal fetal pulmonary vein flow pattern consists of forward flow in systole and diastole, with cessation of flow or a small reversal wave with atrial systole. In a study by Taketazu et al. (69), a pattern of pulmonary vein flow with brief forward and reverse flow with minimal early ventricular diastolic flow was associated with the need for immediate respiratory support and emergent atrial decompression. Two of the three patients with this abnormal flow pattern died after neonatal heart transplantation, and the postmortem lung tissue analysis was notable for dilated lymphatic vessels, pulmonary vein arterialization, and abnormal pulmonary artery musculature.
Fetal Intervention
It is important to remember that most fetuses with severe left ventricular outflow tract obstruction (LVOTO) will survive gestation. Therefore, fetal cardiac intervention in this setting does not serve as a life-saving procedure, but rather a procedure that may improve postnatal surgical options. More specifically, it is hoped that successful intervention in the fetus with LVOTO will lead to a biventricular circulation at the time of birth. This possible benefit must be weighed against the risks of the procedure, which, even in the setting of technical success, may result in fetal death or extreme prematurity. Since the risk/benefit ratio of fetal cardiac intervention in the setting of severe LVOTO is presently unknown, it is not surprising that these procedures are not universally accepted. Some centers have advocated fetal cardiac intervention only when it is felt to be a lifesaving procedure, such as in the setting of critical aortic stenosis with fetal hydrops.
In 2000, Kohl et al. reported the world experience of fetal aortic balloon valvuloplasty (71). The small early clinical experience (n = 12) was quite poor, with only one “long-term” survivor. However, more encouraging data were recently reported by McElhinney et al. (72) from the Children’s Hospital of Boston and the Brigham and Women’s Hospital. These data included 70 fetuses who underwent aortic valvuloplasty for critical aortic stenosis with evolving HLHS between March 2000 and October 2008. There was a significant improvement in technical success (74%), and most procedures were performed with only percutaneous access (73%). Eight fetuses died related to the procedure (11%). Although fetuses with a technically successful valvuloplasty had improved growth of the aortic valve and mitral valve, intervention did not effectively promote left ventricular growth. Therefore, fetuses with larger LV dimensions initially were more likely to sustain a biventricular circulation at the time of birth (n = 15). Based on these results, the authors were able to create a multivariable scoring system, excluding some fetuses who were not likely to respond to fetal intervention.
In utero therapy for HLHS with a severely restrictive or intact atrial septum has also been described. Successful decompression of the left atrium in utero may avoid severe hypoxemia at birth, and theoretically, may also reduce prenatal lung damage and improve otherwise dismal outcomes (73,74,75). In a recent publication by Marshall et al., technically successful atrial septoplasty was performed in 19 of 21 fetuses
between October 2001 and November 2007, with two episodes of fetal death (76). The authors determined that creation of a larger defect was associated with better postnatal oxygenation; however, whether this confers a benefit to later survival is presently unknown.
between October 2001 and November 2007, with two episodes of fetal death (76). The authors determined that creation of a larger defect was associated with better postnatal oxygenation; however, whether this confers a benefit to later survival is presently unknown.
Maternal Hyperoxygenation
In the normal fetus, only a small proportion of the fetal cardiac output is directed to the lungs, with most flow directed across the ductus arteriosus to descending aorta. Studies have demonstrated that maternal hyperoxygenation later in pregnancy can increase pulmonary blood flow, and this has been used to assess pulmonary reactivity in fetuses with suspected pulmonary hypoplasia related to diaphragmatic hernia and severe renal disease (77). In a recent study by Szwast et al., maternal hyperoxygenation was used to assess pulmonary reactivity in fetuses with HLHS and either an open atrial septum or a restrictive/intact atrial septum (78). Mother was administered 100% oxygen via a nonrebreather face mask for 10 minutes, and fetal Doppler assessment of pulmonary artery flow was measured at baseline, with maternal oxygen, and after recovery. The pulsatility index, a surrogate measure of vascular impedance, was used for assessment of pulmonary blood flow. Maternal hyperoxygenation led to a significant increase in pulmonary blood flow in fetuses with an open atrial septum; however, this was not the case in fetuses with atrial septal restriction that required immediate intervention on the atrial septum at birth. It appears that the fetal response to maternal hyperoxygenation is predictive of the need for urgent intervention at the time of birth, and the use of this diagnostic technique can be very helpful when planning the delivery. In the setting of a fetus with HLHS and restrictive or intact atrial septum and lack of pulmonary reactivity, we recommend Caesarean section in either the delivery room or operating room suite so that there can be Immediate Postnatal Access to Cardiac Therapy, either surgical or interventional (IMPACT procedure). The IMPACT procedure was designed to manage high-risk patients and assemble the multidisciplinary resources required to care for these critically ill neonates in the immediate postpartum period (79).
Therapeutic use of maternal hyperoxygenation has also been proposed; as there has also been evidence that chronic intermittent maternal hyperoxygenation in late gestation may cause growth of hypoplastic cardiac structures. Thomas Kohl performed repetitive daily maternal hyperoxygenation in 15 pregnant women between 33 and 38 weeks of gestation (80). The fetal cardiac disease was variable, but 13 of the 15 fetuses had hypoplasia of at least one left heart structure. Kohl demonstrated increases in cardiovascular dimensions (improvements in Z-scores for gestational age) in most fetuses with small ventricles and no inflow/outflow obstruction. The presence of inflow/outflow tract obstruction or a large ventricular septal defect seemed to ameliorate the effect of hyperoxygenation. Maternal hyperoxygenation is a new and exciting potential therapy in selected fetal patients, especially considering its simplicity as well as universal availability. However, the long-term effects of hyperoxygenation on the fetus remain unknown.
Anatomy
Various cardiac malformations characterized by variable degrees of underdevelopment of the left ventricular cavity are referred to as HLHS. Most broadly, HLHS includes any number of lesions with a dominant right ventricle and systemic outflow obstruction that are not amenable to two-ventricle repair.
Underdevelopment of the Left Ventricle Outflow–Aorta Complex
Underdevelopment of the left ventricle outflow–aorta complex, resulting in critical aortic valve stenosis or aortic valve atresia with an intact ventricular septum is the most recognized form of HLHS (81,82,83) (Fig. 46.2). A spectrum of abnormalities includes
aortic valve atresia with mitral atresia, aortic valve atresia with a patent mitral valve, and aortic stenosis with a patent mitral valve. This final group with aortic stenosis and a patent mitral valve blends smoothly into critical aortic stenosis. The general unifying etiologic explanation is that the growth and development of vascular structures are dependent to some degree on the relative quantity of blood flow during fetal development. As mentioned earlier, fetal echocardiographic observations have confirmed the progression from aortic stenosis to HLHS (52). In addition to these observations, supporting data for underdevelopment of the left ventricle outflow–aortic complex as the inciting event are provided by the fact that with an intact ventricular septum, additional lesions, particularly mitral valve hypoplasia, are always less severe than the degree of left ventricular outflow obstruction (84). The ascending aorta is hypoplastic; among patients undergoing surgery for HLHS, the mean aortic diameter was 3.3 ± 1.7 mm; 40% to 55% of patients had an ascending aorta of <2 mm (85,86). Blood flow in the arch is retrograde, and in aortic atresia, the ascending aorta serves only as a conduit for the retrograde flow of blood into the coronary arteries. A localized coarctation of the aorta is present in 80% of patients (9,87). Aortic stenosis with mitral stenosis makes up 23% to 26% of patients undergoing stage 1 palliation, whereas 36% to 46% have aortic atresia with mitral atresia and 20% to 29% have aortic atresia with a patent mitral valve (88,89,90).
aortic valve atresia with mitral atresia, aortic valve atresia with a patent mitral valve, and aortic stenosis with a patent mitral valve. This final group with aortic stenosis and a patent mitral valve blends smoothly into critical aortic stenosis. The general unifying etiologic explanation is that the growth and development of vascular structures are dependent to some degree on the relative quantity of blood flow during fetal development. As mentioned earlier, fetal echocardiographic observations have confirmed the progression from aortic stenosis to HLHS (52). In addition to these observations, supporting data for underdevelopment of the left ventricle outflow–aortic complex as the inciting event are provided by the fact that with an intact ventricular septum, additional lesions, particularly mitral valve hypoplasia, are always less severe than the degree of left ventricular outflow obstruction (84). The ascending aorta is hypoplastic; among patients undergoing surgery for HLHS, the mean aortic diameter was 3.3 ± 1.7 mm; 40% to 55% of patients had an ascending aorta of <2 mm (85,86). Blood flow in the arch is retrograde, and in aortic atresia, the ascending aorta serves only as a conduit for the retrograde flow of blood into the coronary arteries. A localized coarctation of the aorta is present in 80% of patients (9,87). Aortic stenosis with mitral stenosis makes up 23% to 26% of patients undergoing stage 1 palliation, whereas 36% to 46% have aortic atresia with mitral atresia and 20% to 29% have aortic atresia with a patent mitral valve (88,89,90).
There are corresponding changes in the right side of the heart when there is left ventricular cavity hypoplasia. All right-sided cardiac structures are larger than normal including the right atrium, tricuspid valve, pulmonary artery, and pulmonary valve. The right ventricle is both enlarged and hypertrophied (8,9,81,83,87,91,92,93). The anatomy of the interventricular septum may be affected. The apex of the right ventricle and apex of the hypoplastic left ventricle remain in proximity and may be identified externally by the junction of the anterior descending coronary artery and the posterior descending coronary artery. The apical junction of the right and left ventricle will not correspond to the apex of the ventricular mass, because the right ventricle is folded around the hypoplastic left ventricle (94). This may impact tricuspid valve anatomy and function of the right ventricle (95,96).
Abnormalities of the tricuspid valve have been identified in up to 35% of patients with HLHS. In aortic atresia and a patent mitral valve, the left ventricle has inflow but not outflow; the result is significant hypertrophy of a left ventricle that is larger than that with aortic and mitral atresia and in fact may have greater than normal mass (97). The larger ventricular mass may create distortion of the basilar inflow portion of the right ventricle. The septal surface of the right ventricle may appear to have deep apical sinuses or recesses, the result of the apex of the right ventricle folding around the hypoplastic but hypertrophied left ventricle. This distortion of the right ventricle impacts the subvalvar apparatus of the tricuspid valve. The finding of tricuspid valve dysplasia is more common among patients with a patent mitral valve, occurring in 50% in this subgroup (96,98). Tricuspid valve abnormalities can be acquired. Volume overload of the single right ventricle will result in annular dilation and the development of tricuspid insufficiency. Ischemia of the ventricular subendocardium that occurs during the neonatal period either at the time of presentation or following stage 1 palliation will result in chordal elongation with prolapse of the anterior leaflet and the development of tricuspid insufficiency.
Patients with aortic atresia and a patent mitral valve have been reported to be at increased risk for mortality after the Norwood procedure (99,100). Coronary artery abnormalities specifically, fistulous connections between the epicardial coronaries and the left ventricle have been described in a subgroup of these patients (101). EFE is frequently present and is thought to be the result of subendocardial ischemia as a consequence of suprasystemic left ventricular pressure during development (94,97,102,103). Within this subgroup, the potential for tricuspid valve insufficiency is increased and arrhythmias associated with EFE may also contribute to an increased mortality risk (Fig. 46.3) (100).
The least affected subgroup of HLHS is aortic stenosis with a patent mitral valve. This form is thought to develop later during fetal development when the left ventricle is more completely formed. This form blends smoothly into the spectrum of critical aortic stenosis and the borderline left ventricle. Decision-making in patients with left ventricular outflow obstruction can be challenging. In the patient deemed to have a left ventricle that is nonapex forming with a prohibitively hypoplastic mitral valve, stage 1 palliation is commonly chosen with a prognosis that may be favorable because the left ventricle is able to contribute to cardiac output.
Variants of Hypoplastic Left Heart Syndrome
Critical left ventricular hypoplasia may be seen with interrupted aortic arch, critical left ventricular outflow obstruction with a large ventricular septal defect, unbalanced atrioventricular septal defect or double-outlet right ventricle. The presence of a large ventricular septal defect may occur with critical left ventricular outflow obstruction and the absence of suprasystemic left ventricular pressure due to the ventricular septal defect may permit normal development of the left ventricular chamber and mitral valve ultimately permitting biventricular repair. A hypoplastic left ventricle may be present with interrupted aortic arch with a ventricular septal defect but the potential size of the left ventricle in cases of interrupted aortic arch may be difficult to assess in the state of abnormal loading that is present when circulation is dependent on a patent ductus arteriosus. A prohibitively small left ventricle can occur with unbalanced atrioventricular septal defects but again the potential size of the left ventricle may be difficult to determine with abnormal loading conditions. Profound hypoplasia of the left ventricle is seen in double-outlet right ventricle with mitral atresia and this can occur even without obstruction to aortic outflow suggesting that inflow starvation can result in HLHS.
Additional Anatomic Considerations
Abnormalities of systemic venous return are uncommon in the patient with HLHS. Persistent left superior vena cava (SVC) occurs in about 15% (8,83). Abnormal pulmonary venous drainage occurs in about 5% of patients (104). An intact or highly restrictive atrial septum occurs in about 1% of neonates with HLHS and in about half of these the atrial septum is imperforate. Occasionally there is a persistent levocardinal vein that drains to the innominate vein and allows for atrial decompression (105). As discussed previously an intact atrial septum can lead to pulmonary vascular changes in utero and these changes persist despite successful postnatal atrial septal defect creation and result in a high mortality in this group. Fetal intervention may limit or allow reversal of these changes in the pulmonary vasculature. Rare cases of anomalous pulmonary venous connection directly to the right atrium also exist.
Important coronary abnormalities are rare. Anomalous origin of either of the coronary arteries from the right pulmonary artery has been described (106,107,108,109). Coronary-cameral fistulas have been observed in patients with aortic atresia and a patent mitral valve. Additionally, patients with aortic atresia and a patent mitral valve have been observed to have tortuous epicardial coronaries with increased medial thickness (110). Despite the origin of the coronary arteries from the small ascending aorta, the coronary ostia and proximal coronary artery calibers are normal (111).
Premature closure of the patent foramen ovale has been postulated as a cause of HLHS. Because the foramen ovale is the source of left ventricular preload in the fetus, one would expect that closure of the foramen ovale would starve the left ventricle of preload and result in hypoplasia. Premature closure of the foramen ovale may also occur as a secondary event to left ventricular outflow obstruction. As mentioned earlier, LVOTO will result in increased
left atrial pressure, and increased left atrial pressure results in apposition of the fossa ovalis flap valve against the septum secundum. Premature closure or restriction of the foramen ovale might occur along with LVOTO and contribute to the development of HLHS. Among patients with an intact ventricular septum, premature closure of the foramen ovale was associated with EFE and indicates that LVOTO with elevated left ventricular end-diastolic pressure and subendocardial ischemia was present (112). Among patients with premature foraminal closure and a ventricular septal defect, fibroelastosis was absent, indicating that premature closure was perhaps a primary event in the development of left ventricular hypoplasia.
left atrial pressure, and increased left atrial pressure results in apposition of the fossa ovalis flap valve against the septum secundum. Premature closure or restriction of the foramen ovale might occur along with LVOTO and contribute to the development of HLHS. Among patients with an intact ventricular septum, premature closure of the foramen ovale was associated with EFE and indicates that LVOTO with elevated left ventricular end-diastolic pressure and subendocardial ischemia was present (112). Among patients with premature foraminal closure and a ventricular septal defect, fibroelastosis was absent, indicating that premature closure was perhaps a primary event in the development of left ventricular hypoplasia.
The Borderline Left Ventricle
Borderline left ventricle is not a specific anatomic subtype of HLHS but rather a group of lesions with patent mitral and aortic valves that in past may have been submitted for staged single-ventricle palliation and that are today the subject of efforts to achieve a two-ventricle repair. The conversion to two-ventricle repair may take place in the neonatal period or at a later stage. Among those subjected to early conversion the hypoplasia is primarily in the left ventricular outflow tract. EFE is common and resection of EFE is performed along with mitral valve repair. While there may be mitral stenosis, the mitral valve annular dimensions are typically within the normal range (113). Efforts to achieve late conversion are centered on creating hemodynamic lesions, specifically elevated left atrial pressure in order to promote catch up growth of the left ventricular inflow and left ventricular cavity. These procedures often include a Norwood with a restrictive atrial septal defect that is followed up by a second-stage operation that combines a bidirectional Glenn shunt with EFE resection, mitral valvuloplasty, a restrictive atrial septal defect, and construction of an additional source of pulmonary blood flow again to create above normal left atrial pressure to promote growth of the mitral valve and left ventricle. The results thus far suggest a successful conversion of around one-third but the variability of the starting anatomy and relatively limited outcomes measure (transplant free survival only) makes interpretation of this treatment strategy difficult (114).
Presentation, Diagnosis, and Echocardiographic Imaging
Presentation
Today, many cases of HLHS are detected in the second trimester when a screening obstetric ultrasound shows an abnormal four-chamber view. Prenatal recognition of the disease allows timely parental counseling as well as optimal delivery planning. Delivery at a tertiary care facility is recommended, avoiding transport-related morbidities, and allows the mother to be in close proximity to her baby after birth (115). Most centers continue to advocate a vaginal delivery, although induction of labor may be deemed necessary if the mother lives a significant distance from the tertiary care facility. Following delivery, prostaglandins are initiated to maintain ductal patency, and an echocardiogram is performed to confirm the diagnosis. If severe atrial septal restriction is suspected on the prenatal ultrasound, interventional cardiology and/or cardiothoracic surgery should be immediately available.
If the infant has not been prenatally diagnosed with HLHS, timing of presentation is somewhat variable and dependent on the degree of atrial-level restriction as well as ductal patency. During late fetal development, pulmonary vascular resistance (PVR) is high and pulmonary blood flow is limited to <10% of ventricular output. At birth, PVR decreases abruptly as a result of mechanical distention of the lung, increased oxygen tension, and increased shear stress. Although the greatest fall in PVR occurs shortly after birth, a clinically important decline in PVR continues within days of birth (116,117,118). Abu-Harb et al. (119) reviewed the time of presentation of obstructive left heart malformations. About one-quarter of their infants with HLHS became symptomatic within 24 hours of age. However, most infants had a “normal” neonatal examination, with development of symptoms after 48 hours of age, often after hospital discharge. When the atrial septum is restrictive, the resultant left atrial hypertension leads to pulmonary congestion, resulting in early onset of tachypnea and cyanosis. When the atrial septum is widely patent, neonates with HLHS may initially appear normal, with adequate oxygenation and systemic perfusion. These infants have a more delayed presentation; with symptoms developing as the ductus arteriosus undergoes gradual spontaneous closure. With ductal regression, there is hypoperfusion of the systemic circulation with an associated augmentation of pulmonary blood flow. These infants present at 2 to 3 days of age with feeding difficulties and respiratory distress, with rapid progression to congestive heart failure and shock.
The survival benefit of prenatal diagnosis on surgical outcomes for HLHS is reported with mixed findings (120,121,122). Prenatal diagnosis alone has also not been found to reduce preoperative neonatal mortality. However, postnatal diagnosis along with greater distance from the receiving cardiac surgical center or obstructed pulmonary venous return or a major extracardiac congenital abnormality is linked to greater preoperative death (123,124). Importantly, prenatal diagnosis can be protective against white matter injury and may in fact be associated with less need for preoperative respiratory and circulatory support (125).
Diagnosis
Physical examination in the neonate with a severely restrictive atrial septum will be most notable for intense cyanosis with respiratory distress. In contrast, the infant with a nonrestrictive atrial defect may appear relatively pink. The infant with ductal closure is often lethargic and has respiratory distress, cool extremities, and pallor. Auscultation is generally benign, especially in comparison with a sometimes dramatic clinical picture. The second heart sound is single and loud, reflecting the absence of the aortic valve component and the associated pulmonary artery hypertension. A third heart sound may be heard, especially in the presence of ventricular dysfunction. Murmurs are uncommon, although a soft systolic ejection murmur may be generated from increased flow across the pulmonary valve. A louder S1 coincident murmur may be heard if there is significant tricuspid regurgitation. The upper- and lower-extremity pulses are palpable and symmetric early but are reduced later as ductal closure ensues. Hepatomegaly is common and is generally seen in infants with a delayed presentation.
Chest radiographs are generally nondiagnostic but typically reflect the degree of atrial-level restriction. In the infant with a severely restrictive atrial septum, the heart size may be relatively normal; however, there is significant pulmonary edema. The radiographic findings may be misinterpreted as lung disease, leading to a delay in diagnosis. In contrast, if the atrial septum is nonrestrictive, there is pulmonary overcirculation with cardiomegaly. The right atrial border may be prominent with absence of the ascending aortic shadow.
The electrocardiogram does reflect the underlying pathology; however, it is nondiagnostic. Right axis deviation and right ventricular hypertrophy are common, but not distinctly different from the normal electrocardiogram of the neonate. Tall, peaked p waves, indicative of right atrial enlargement, have been reported in 30% to 40% of patients (7,8).
With diagnostic two-dimensional echocardiography readily available, the need for cardiac catheterization in an infant with HLHS has dramatically decreased. Cardiac catheterization is generally used as an adjunct tool when trying to better identify pulmonary venous anomalies, or possibly, coronary anomalies. Also, in the setting of a severely restrictive atrial septum, catheter intervention may be lifesaving.
Echocardiography
The diagnosis of HLHS can be readily made by two-dimensional echocardiography, with additional important hemodynamic information provided by Doppler echocardiography (104,126,127,128,129,130,131,132,133). The intracardiac anatomy and physiology should be investigated using a standard echocardiographic approach and should include multiple imaging views (long-axis, short-axis, apical four-chamber, subcostal coronal, subcostal sagittal, suprasternal notch) with repeated Doppler assessments.
Parasternal Long-Axis View
The diagnosis of HLHS is often suspected immediately from a parasternal long-axis view with identification of a small, muscle-bound left ventricular chamber that does not extend to the cardiac apex (Fig. 46.4). The endocardial surface of the left ventricle is often echo-bright, indicating areas of EFE. The left atrium is usually small but may be dilated in patients with a restrictive atrial septal defect. The ascending aorta can be well visualized from the long-axis view and is frequently small (2 to 3 mm in diameter); the aortic valve may or may not be patent. The mitral valve is often imperforate, but when patent, the leaflets are thickened, with short or even absent papillary muscle chordal attachments. A ventricular septal defect is rare in the presence of aortic atresia, but color Doppler interrogation of the ventricular septum may show ventriculo-coronary arterial connections. Although the significance of these abnormal coronary connections in HLHS is unclear, coronary artery sinusoidal connections have had prognostic implications in other forms of CHD (134,135).
Several measurements are available from the parasternal long-axis view, which can be helpful when trying to differentiate critical aortic stenosis from HLHS. A left ventricular cross-sectional area <1.5 cm2 is found in most infants with HLHS, as well as a left
ventricular end-diastolic inflow dimension <25 mm (measured from the hinge point of the posterior mitral leaflet to the apex) and a mitral annulus diameter of ≤6 mm (126,127).
ventricular end-diastolic inflow dimension <25 mm (measured from the hinge point of the posterior mitral leaflet to the apex) and a mitral annulus diameter of ≤6 mm (126,127).
Parasternal Short-Axis View
The parasternal short-axis view again allows assessment of left ventricular size and function (Fig. 46.5A). The mitral valve papillary muscles are well visualized from this window and should be carefully examined. At the base of the heart, aortic valve size and anatomy can be well visualized. Doppler interrogation of the coronary arteries is often best assessed here. Bidirectional coronary flow is consistent with left ventriculo-coronary arterial connections. Finally, the main pulmonary artery, pulmonary valve, and branch pulmonary arteries are all well seen from the short-axis view. Scanning more superiorly, the patent ductus arteriosus can be visualized as it sweeps to the descending aorta (Fig. 46.5B).
Apical Four-Chamber View
The apical four-chamber view is often critical for definitively evaluating left ventricular size and function. If a large portion of the cardiac apex is occupied by the right ventricle (Fig. 46.6), it is unlikely that the left ventricle can support the systemic circulation. The four-chamber view also provides an excellent window to see the entire mitral apparatus, including the subvalvar and supravalvar areas. Mitral valve anatomy and annulus size should be reassessed, especially in cases of borderline left ventricular size.
Right ventricular function and tricuspid valve anatomy and competency are best assessed from the four-chamber view. Right ventricular systolic function may be depressed, especially in those neonates with ductal closure and acidosis. Tricuspid valve abnormalities are common and can include a bileaflet valve, tricuspid valve dysplasia/prolapse, and abnormal papillary muscle arrangements (96).
Subcostal Views
Atrial septal anatomy is best imaged from the subcostal views. Large atrial septal aneurysms billowing into the right atrium are common (Fig. 46.7). Unusual attachments of septum primum can sometimes be seen, specifically anomalous attachment to the posterosuperior left atrial wall (Fig. 46.8). This anomalous attachment has been implicated in the pathogenesis of HLHS (59). If the atrial defect is small and restrictive, peak and mean Doppler gradients across the atrial septum should be obtained to estimate the degree of left atrial hypertension.
Pulmonary venous anatomy and drainage should also be interrogated from the subcostal window. It is important to identify connections of the pulmonary veins (anatomic attachment) as well as pulmonary venous drainage (the end point of pulmonary venous flow). The pulmonary veins may connect normally to the left atrium, but especially in cases of an intact atrial septum, there may be a levoatrial cardinal vein that originates directly from the left atrium and drains either all pulmonary veins (total) or some (partial) to a variable location. It is important to remember that this anomalous venous structure can be stenotic, so the presence of the “decompressing” vein does not guarantee normal left atrial pressure (74). On the other hand, some or all of the pulmonary veins may not connect normally to the left atrium, but connect to a confluence behind the left atrium with anomalous drainage to a variable location. It is estimated that anomalous pulmonary venous anatomy and/or drainage occurs in about 5% to 10% of patients with HLHS.
Suprasternal Notch Views
The suprasternal notch provides an important window for evaluating aortic arch anatomy (Fig. 46.9). Although the ascending aorta can be imaged from many views, the transverse arch and descending thoracic aorta are best seen from the suprasternal notch view. Coarctation of the aorta is common in patients with HLHS, and interruption of the aortic arch has also been reported. Doppler interrogation of the transverse arch should show retrograde systolic flow from the ductus; this finding indicates ductal-dependent systemic circulation and supports left ventricular inadequacy for biventricular repair (Fig. 46.10). The suprasternal notch views also provide images of the proximal pulmonary arteries and the ductus arteriosus. In the patient with a later presentation, the ductus may be restrictive; Doppler interrogation of the pressure gradient from pulmonary artery to aorta should be quantified and follow-up studies performed when prostaglandin therapy is initiated. Pulmonary venous connection and drainage should be reassessed from this window. A persistent left SVC or levoatrial cardinal vein can be well imaged to the left of the descending aorta from the suprasternal notch view.
Physiology, Monitoring, and Stabilization
Parallel Circulation
The patient with HLHS faces similar physiologic challenges before, during, and after stage 1 palliation. The superimposition of inefficient parallel circulation, cyanosis, myocardial dysfunction, and
autonomic and inflammatory responses to stress and surgery result in high likelihood of critical impairment of oxygen delivery (DO2) with subsequent organ dysfunction or death. Thus, facility with the principles of hemodynamics and oxygen supply/demand economy is a prerequisite for rational perioperative treatment of first-stage palliation patients.
autonomic and inflammatory responses to stress and surgery result in high likelihood of critical impairment of oxygen delivery (DO2) with subsequent organ dysfunction or death. Thus, facility with the principles of hemodynamics and oxygen supply/demand economy is a prerequisite for rational perioperative treatment of first-stage palliation patients.
Maintenance of adequate organ substrate (oxygen) delivery is necessary to reverse or prevent ischemic injury, which can result in multisystem organ dysfunction, prolonged morbidity, and mortality (136,137,138,139,140,141,142). Interventions targeting early treatment of inadequate whole-body or regional oxygen supply/demand relationships (shock) have improved outcome in critical illness; therefore detection of inadequate DO2 is important for preventive or therapeutic interventions (136,137,143,144,145,146,147,148).
Cardiovascular Reflexes and Physiology of Shock
Local, regional, and global circulatory control mechanisms exist to optimize and prioritize efficient DO2 to meet metabolic demand. Global cardiac output is affected by preload, afterload, rate, rhythm, contractility, and the presence of aortopulmonary shunts. Regional resistance is determined by the interaction of neurohumoral factors related to inflammation and the sympathetic nervous system, and local factors related to autoregulation. The total systemic vascular resistance (SVR) is thus determined by the net effect of regional resistances. DO2 is systemic cardiac output (Qs) multiplied by arterial oxygen content (CaO2), which is determined by the hemoglobin (Hb) concentration, oxygen saturation (SaO2), and oxygen tension (PaO2):


The sympathetic stress response as described with hypovolemic-septic shock (149,150,151) is activated to redistribute available blood flow to the brain and heart (152,153,154). The distribution of cardiac output will thus be significantly altered by sympathetic responses activated in all shock states, with the mesenteric and splanchnic circulations being at high risk for ischemia which may be unrecognized during nonhypotensive (compensated) shock (155,156,157,158). Circulatory reflexes to hemorrhage or hypotension will increase baroreflex gain to raise contractility, heart rate, and SVR, and decrease venous capacitance (159,160,161,162). These responses may be immediately protective in the face of hemorrhagic shock but often impair systemic flow in the face of myocardial dysfunction (163,164). These responses are also activated by cold stress, pain, and anxiety, and thus are not specific to hypovolemia (165,166,167,168). The vigor of the vascular component of the stress response may actually cause blood pressure to be elevated in the face of low cardiac output in the stressed neonate or child (169). The predominant profile of shock in pediatric patients is low cardiac output and very high SVR (170).
The vulnerability of the splanchnic organs to ischemic injury results from intense sympathetic innervation, regional density of alpha-adrenergic receptors (157,171,172,173,174), and selective effects of angiotensin (175,176). Ischemic organ damage may occur even in the presence of normal global oxygen economy if regional vascular resistance is sufficiently elevated (155,156,177,178,179). There now exists compelling evidence that splanchnic/mesenteric ischemia is a frequent common pathway for multisystem organ dysfunction and death (180,181,182,183), and regional cellular oxygen deficit is underrecognized, underdiagnosed, and undertreated (184). Strategies targeting earlier detection and treatment of shock could improve outcome, with greater impact in populations with higher baseline mortality risk (185). The mortality risk in the neonate with HLHS, even with contemporary management, is high enough to justify the additional complexity of oxygen-delivery goal-directed approaches to prevention, detection, and management of shock states (186).
Oxygen Flux in Single-Ventricle Parallel Circulation
An important limitation in circulatory physiology in the patient with univentricular mixing circulation is that increases in systemic oxygen consumption (VO2) cause a decrease in DO2, and therefore metabolic demands cannot be met by increased extraction, but only by increased systemic DO2 (187). This limitation is best clarified by
comparison of the oxygen cascade diagram between in-series and parallel circulation (Fig. 46.11).
comparison of the oxygen cascade diagram between in-series and parallel circulation (Fig. 46.11).
In a patient with normal in-series circulation, an increase in VO2 does not directly change arterial DO2, and the increased demand may be met by a combination of increases in extraction or DO2. At constant cardiac output, increased VO2 will reduce SvO2, but pulmonary oxygen uptake will increase to match. In the critically ill patient, tissue oxygen utilization will usually continue until the SvO2 falls to <50%; thus, a doubling of VO2 can be met without an increase in cardiac output. Since normal lungs can fully oxygenate fully desaturated systemic venous blood, the resulting SaO2 is unchanged, DO2 is maintained, and the increased VO2 can be met by increased extraction alone. Similarly, cellular oxygen utilization can be maintained during a reduction in cardiac output and DO2 by increased extraction. Thus short-term or moderate increases in VO2 do not require increased cardiac output.
In a patient with univentricular parallel circulation, increased oxygen demand will result in an immediate decrease in oxygen delivery. Any increase in oxygen extraction (either because of increased VO2 or decreased DO2) will reduce SvO2 and therefore SaO2. The result is that conditions that increase oxygen extraction will also decrease oxygen delivery through a reduction in SaO2. For any given Qp/Qs, the increased tissue oxygen demand can be met only by increased cardiac output. For any given fall in cardiac output, DO2 and SvO2 will be disproportionately reduced, because SaO2 will also fall. Thus, changes in VO2 are inversely linked to oxygen supply, and increases in metabolic demands are inherently destabilizing in the patient with parallel univentricular physiology.
With univentricular parallel anatomy, both the pulmonary circulation and the systemic circulation are fed by arterial blood that is only partially saturated with oxygen. Because arterial saturation (SaO2) is reduced by mixing, and because Qs may be reduced by various factors, a reduction in DO2 is typically encountered in patients with HLHS, with a resulting higher risk of cellular hypoxia as a major physiologic vulnerability. Applying the Fick principle (equality of systemic VO2 and pulmonary oxygen uptake) to the patient with HLHS yields the following relationships between VO2, pulmonary blood flow (Qp), systemic blood flow (Qs), SaO2, systemic venous saturation (SvO2), and pulmonary venous saturation (SpvO2), which allows estimation of the pulmonary-to-systemic flow ratio (Qp/Qs) in the parallel circulation:



Solution of the Fick equation for SvO2 shows an intuitive relationship to SaO2 and the oxygen demand/supply ratio:

However, in single-ventricle parallel circulation, the SaO2 depends on both systemic and pulmonary flow and venous saturation:

These complex interactions require estimation of not only SaO2, but also SvO2 and SpvO2 to characterize the adequacy and efficiency of parallel circulation. These equations have been generalized for a continuous range of systemic and pulmonary mixing, allowing application to patients with partially preserved left ventricular transitional circulation (188).
Optimal systemic oxygen delivery in univentricular models occurs at the lowest total cardiac output when Qp/Qs is close to 1 (189). This economy occurs with the total ventricular output (Qt) being twice the normal output of an in-series systemic ventricle, to yield normal values for both Qs and Qp. With a Qp/Qs of 1 and an arterial-venous saturation difference (SaO2 − SvO2) of 25%, oxygen uptake/consumption equilibrium will occur when the pulmonary capillary–arterial saturation difference (SpvO2 − SaO2) also equals 25%, resulting in an SaO2 of 75% and an SvO2 of 50%, assuming that pulmonary venous blood is fully saturated. If SaO2 is >75%, a higher Qp is necessary to maintain the same pulmonary O2 uptake; conversely if Qp falls, SaO2 will also fall. If the SaO2 is low, then a higher Qs is necessary to maintain systemic O2 uptake; if Qs falls, then SaO2 also falls. Changes in SaO2 result in opposite effects on pulmonary and systemic oxygen economy. Conversely, since a tradeoff of Qs and Qp will exist for any Qt, increases in SaO2 that are not a result of increased Qt will be offset by a reduction in Qs. Referring to the first three examples in Table 46.1, moderately large changes in Qp/Qs with constant Qt (assuming a hemoglobin of 15 g/dL, SpvO2 of 100%, and indexed VO2 of 160 mL/m2/min) show a range of SaO2 from 63% to 82% but a narrower range of SvO2 for 44% to 50%. As a result, moderate alterations in Qp/Qs balance will have minimal effect on DO2; more effectively alterable determinants of DO2 include hemoglobin and Qt. Oxygen economy at higher or lower Qp/Qs and varying Qt is illustrated in Table 46.1.
Changes in Qt, over a range of Qp/Qs, have a more profound effect on SvO2. Thus matching of DO2 to changes in VO2 is more effective via interventions in total cardiac output or hemoglobin concentration than by precise manipulation of Qp/Qs balance.
Monitoring the Parallel Circulation
The first successful approaches to monitoring and managing the patient with HLHS emphasized the central importance of SaO2 in detecting and guiding treatment of unbalanced pulmonary-to-systemic blood flow ratio and total cardiac output (190). Application of this approach was based on extrapolation from circulatory models that assumed either a constant arteriovenous oxygen difference (of typically 25%) or a constant mixed SvO2 (of typically 50%). In either model, an SaO2 of 75% would then result from mixing equal parts systemic venous and (fully saturated) pulmonary venous blood; deviations of SaO2 from 75% in these models would result from, and be diagnostic of, deviations of Qp/Qs from 1. These approaches also assumed adequate total cardiac output to meet oxygen delivery needs if Qp/Qs is optimized. Under these conditions, systemic oxygen delivery generally increases as SaO2 increases toward 75% to 80%, and falls at higher SaO2 owing to increasing Qp/Qs imbalance. However, in the perioperative period, total cardiac output and metabolic
demand may frequently be mismatched as a result of the inherent instability of parallel circulation as described above, and variability of Qp/Qs, Qt, and VO2 (191,192,193). Inspection of Table 46.1 reveals that assertions about the Qp/Qs from a single measured SaO2 value are unreliable unless either SvO2, or VO2 and Qt, are known. A target SaO2 of 75% can result from a range of Qp/Qs and SvO2 conditions, which may include inadequate systemic flow if Qt or VO2 is variable. In a circulatory model that allows variation in both total cardiac output and Qp/Qs, a wide range of tissue/venous saturation can result at any given SaO2, shown graphically in Figure 46.12. The resulting domain of SvO2 shows that severely impaired systemic oxygen delivery can occur with SaO2 closely maintained in the target 75% to 80% range.
demand may frequently be mismatched as a result of the inherent instability of parallel circulation as described above, and variability of Qp/Qs, Qt, and VO2 (191,192,193). Inspection of Table 46.1 reveals that assertions about the Qp/Qs from a single measured SaO2 value are unreliable unless either SvO2, or VO2 and Qt, are known. A target SaO2 of 75% can result from a range of Qp/Qs and SvO2 conditions, which may include inadequate systemic flow if Qt or VO2 is variable. In a circulatory model that allows variation in both total cardiac output and Qp/Qs, a wide range of tissue/venous saturation can result at any given SaO2, shown graphically in Figure 46.12. The resulting domain of SvO2 shows that severely impaired systemic oxygen delivery can occur with SaO2 closely maintained in the target 75% to 80% range.
TABLE 46.1 Effect of Varying Qp/Qs and Qt on Arteriovenous Saturations in Parallel Circulation | ||||||||||||||||||||||||||||||||||||||||||||||||||||||||||||||||||||||||||||||||||||||||
---|---|---|---|---|---|---|---|---|---|---|---|---|---|---|---|---|---|---|---|---|---|---|---|---|---|---|---|---|---|---|---|---|---|---|---|---|---|---|---|---|---|---|---|---|---|---|---|---|---|---|---|---|---|---|---|---|---|---|---|---|---|---|---|---|---|---|---|---|---|---|---|---|---|---|---|---|---|---|---|---|---|---|---|---|---|---|---|---|
|
An early report of increased clinical stability with the use of inspired carbon dioxide (CO2) (187) led to the wide adoption of manipulation of medical gases to control PVR and Qp/Qs. Theoretic and experimental models showed that inspired CO2 increased PVR, and moderately decreased SVR, and would increase systemic DO2 (194,195). As part of this approach, the SaO2 was used as a key indicator to detect pulmonary overcirculation, which would result in a higher SaO2 as Qp/Qs rose. However, this would be true only if the systemic arteriovenous difference did not increase, which would occur only if the increase in Qp resulted from increased Qt at constant Qs. The primary concern over preventing a runaway spiral of increased Qp/Qs led to the use of low or even subatmospheric fraction of inspired oxygen (fiO2) in further attempts to raise the PVR (196,197).
The unrestricted pulmonary-to-systemic flow in the preoperative patient with ductal patency maintained with prostaglandin creates a period of increasing physiologic vulnerability (125,198) as PVR falls in the hours and days after birth. Preoperatively, manipulation of alveolar gas tensions to modulate PVR may be partially effective in limiting pulmonary overcirculation, but only hypercapnia increases systemic and or cerebral oxygen delivery (199). Use of alveolar gas management strategies in the preoperative period are associated with worse outcomes (200,201,202). In contrast, a synthetic shunt placed at the time of initial palliation imposes a large fixed resistor into the total pulmonary resistance, which is optimally of similar magnitude to the SVR. This arrangement reduces the efficacy
of PVR manipulations on hemodynamics (203,204). Reduction of fiO2 may cause the resulting alveolar oxygen tension to be inadequate to fully oxygenate the pulmonary capillary blood, an effect that may be common at fiO2 <0.3 (205). Thus, reduction in SaO2 by intentionally limiting fiO2 may result solely from pulmonary capillary desaturation rather than reductions in Qp. This will reduce oxygen uptake across the lung, waste pulmonary blood flow, and reduce oxygen available for tissue utilization. Unless SpvO2 is measured or fiO2 is high enough to make pulmonary capillary desaturation unlikely, the calculated Qp/Qs at low fiO2 may be falsely low because of SpvO2 <95% (205). Because of variability in both SpvO2 and the arteriovenous saturation difference, the SaO2 does not reliably characterize the parallel circulation.
of PVR manipulations on hemodynamics (203,204). Reduction of fiO2 may cause the resulting alveolar oxygen tension to be inadequate to fully oxygenate the pulmonary capillary blood, an effect that may be common at fiO2 <0.3 (205). Thus, reduction in SaO2 by intentionally limiting fiO2 may result solely from pulmonary capillary desaturation rather than reductions in Qp. This will reduce oxygen uptake across the lung, waste pulmonary blood flow, and reduce oxygen available for tissue utilization. Unless SpvO2 is measured or fiO2 is high enough to make pulmonary capillary desaturation unlikely, the calculated Qp/Qs at low fiO2 may be falsely low because of SpvO2 <95% (205). Because of variability in both SpvO2 and the arteriovenous saturation difference, the SaO2 does not reliably characterize the parallel circulation.
The importance of both SVR and PVR in determining Qp/Qs was emphasized in modeling studies (203). In these studies, the Qp/Qs range could be restricted by placement of a resistive shunt, and the importance of shunt size was emphasized. These models also demonstrated that the combination of low total cardiac output and high Qp/Qs severely impaired systemic oxygen delivery. Even in the presence of a resistive shunt in the pulmonary circulation, control of elevated SVR was more effective than increases in PVR to optimize systemic oxygen delivery.
Although noninvasive, continuous, and nearly universally available, pulse oximetry only estimates SaO2, which does not measure DO2 or its adequacy. Not surprisingly, perioperative management based primarily on optimization of SaO2 is associated with an early mortality of >20%. With this approach, cardiovascular collapse and mortality typically result from an acute hemodynamic event that occurs unexpectedly in an apparently stable postoperative hemodynamic setting (88,206,207). Without measurement of SvO2 or tissue oxygenation, the effects of treatment, pathology, or state-related changes in patient physiology on systemic DO2 must be inferred from physical examination and complex pattern recognition in heart rate, blood pressure, and arterial saturation, which are nondiagnostic, and intermittent laboratory assessment, which is diagnostic only in retrospect (208).
Because sympathetic vasomotor tone and thus SVR increase as systemic flow falls, changes in Qp/Qs can occur rapidly, resulting in deterioration of systemic DO2 in a self-reinforcing cycle. Precisely because of the Qp/Qs tradeoff, these changes are usually not readily apparent with arterial pressure or oxygen saturation monitoring as demonstrated in Figure 46.13. This above analysis provides an explanation for the profound circulatory derangements that are possible despite having SaO2 in the typical target range. These theoretical and actual limitations have led to the development of management strategies aided by SvO2 measurement to more closely assess Qp/Qs, adequacy of DO2, and whole-body oxygen economy.
It is not possible to obtain a true mixed venous blood sample in the patient with HLHS, but approximate measures of the mixed venous oxyhemoglobin saturation can be obtained from blood withdrawn from the SVC. Placement of a catheter in the SVC primarily to allow sampling of quasi-mixed venous blood is more likely to be helpful in guiding perioperative management. Small (4 Fr) oximetric catheters placed in the SVC just prior to weaning
from bypass in neonates undergoing Norwood-type repairs allow continuous readout of SVC saturation. Used as an approximation of SvO2, monitoring of SVC saturation allows timely hemodynamic intervention to avoid anaerobic metabolism, which has an apparent SVO2 threshold near 30% in this population (209). The use of continuous SVO2 has greatly reduced the perioperative occurrence of sudden unexpected circulatory collapse (88,206,210). However, this measure is available only rarely preoperatively, and for a limited time postoperatively.
from bypass in neonates undergoing Norwood-type repairs allow continuous readout of SVC saturation. Used as an approximation of SvO2, monitoring of SVC saturation allows timely hemodynamic intervention to avoid anaerobic metabolism, which has an apparent SVO2 threshold near 30% in this population (209). The use of continuous SVO2 has greatly reduced the perioperative occurrence of sudden unexpected circulatory collapse (88,206,210). However, this measure is available only rarely preoperatively, and for a limited time postoperatively.
Near-infrared spectroscopy (NIRS) monitoring of tissue oxygen status has been used as an adjunct to perioperative management. NIRS devices with a 4-cm source-detector distance can measure the average oxyhemoglobin saturation (rSO2) in tissue about 2 to 3 cm below the skin, and has been used to monitor oxyhemoglobin saturation in a range of organs including brain, muscle, kidney, and gut (211,212). The probes are most commonly placed on the forehead to monitor cerebral oxygenation, and on the T10–L2 flank region to monitor somatic saturation, as an attempt to capture circulations under intense autoregulatory (cerebral) and autonomic (renal or splanchnic somatic) control. Combining NIRS rSO2 from these two regional circulations in a linear model allows better approximation of SvO2; thus it provides information about both regional and global oxygen economies as a noninvasive and continuous SvO2 surrogate (213,214,215,216) (Fig. 46.14). Given the instability of oxygen supply/demand relationships, and the inadequacy of assessment based on arterial blood pressure and SaO2 monitoring, improved outcome requires early detection and treatment of deficiencies in oxygen economy. Direct or surrogate measurement of SvO2 permits continuous assessment of adequacy of systemic DO2 in the most vulnerable postoperative period. Low total cardiac output and unbalanced Qp/Qs can be differentiated physiologically, interventions can be rationally based, and patient responses can be quantified and trended. We have found continuous venous-side monitoring (SVC SvO2 monitoring or cerebral and somatic NIRS) to be the most important factor in maintaining high survival after stage 1 palliation (88), and our current approach relies on noninvasive pre- and postoperative detection of circulatory deterioration with NIRS (202,217,218).
Preoperative Preparation
Prostaglandin E1
Maintaining ductal patency in a neonate with HLHS is vital in the preoperative management. Although ductal closure is rarely immediate, nearly all infants will have physiologic closure of the ductus arteriosus by the fourth day of life; 20% of infants will demonstrate functional ductal closure during the first day of life, and >80% of infants demonstrate ductal closure during the second day of life. For this reason, prostaglandin E1 (PGE1) therapy should be initiated immediately when HLHS is diagnosed or suspected (219,220,221). The patient’s physiologic state often directs initial PGE1 dosing. For patients who present in shock with suspected ductal closure or a restrictive duct, initial dosing will range from 0.05 to 0.1 μg/kg/min. Once ductal patency is ensured, the infusion rate can be decreased to an effective dose as low as 0.01 μg/kg/min (222).
Ductal patency with the lowest effective PGE1 dose minimizes the most common dose-dependent side effects of PGE1: hypotension prompting volume resuscitation and respiratory depression requiring mechanical support (222,223,224). Initiation of intravenous caffeine with a loading dose of 20 mg/kg followed by a maintenance dose of 5 to 10 mg/kg/day has been effective in reducing the need for mechanical ventilation preoperatively. This approach is based on a study in neonates receiving low-dose PGE1 while simultaneously being treated with aminophylline or placebo. Patients who received aminophylline had a decreased incidence of apnea and did not require intubation when compared with the placebo group of whom 35% required intubation (225).
Respiratory Support and Inspired Gases
In the preoperative patient without anatomic limitation to pulmonary blood flow, mechanical ventilation and medical gas manipulation of pulmonary arteriolar resistance are sometimes necessary and beneficial. Controlled positive-pressure ventilation with care taken to avoid hyperventilation can limit pulmonary blood flow. Additionally, the use of positive end-expiratory pressure (PEEP) allows delivery of lung volumes that exceed functional residual capacity and subsequently results in compression of pulmonary vasculature with a resultant increase in PVR.
Medical gas management can be used to increase PVR and reduce Qp/Qs. Inspired CO2 as a means to limit pulmonary blood flow and treat instability first became apparent in the early 1990s (190). Subsequent animal models have confirmed the effectiveness of inspired CO2 on lowering the pH and raising PVR while successfully increasing systemic flow (194,195,226). Animal models have similarly demonstrated the effectiveness of subatmospheric fiO2 in increasing PVR (195,226). Clinical experience supports the use of hypoxia as a means to attenuate an elevated Qp/Qs (196,227). Hypoxic gas mixtures are achieved through blending nitrogen and oxygen to achieve inspired subatmospheric fiO2 of 0.14 to 0.20. The use of inspired gases in humans has been best studied in an acute model by Tabbutt et al. (199). Preoperative neonates with HLHS who were anesthetized and under neuromuscular blockade were ventilated with a hypoxic gas mixture (fiO2 of 0.17) and with supplemental CO2 (fiCO2 of 0.03). Although both strategies were successful in acutely reducing SaO2 and Qp/Qs, only hypercarbia improved systemic oxygen delivery (199). Furthermore, whereas hypercarbia improved cerebral oxygenation, hypoxia provided no benefit to cerebral saturation (228).
In general, excessive supplemental oxygen, a potent pulmonary vasodilator, is avoided in the preoperative management of HLHS, although patients with respiratory distress syndrome, pneumonia, atelectasis, or other primary lung pathology might benefit from the use of supplemental oxygen for severe hypoxia. Patients with restrictive atrial communication also necessitate supplemental
oxygen administration. For these patients, controlled ventilation is instituted with supplemental oxygen, with titration based upon both arterial and regional or venous oxygen measures by NIRS, which allows the decoding of the complex interactions of fiO2 and pCO2 on pulmonary blood flow, systemic blood flow, and total oxygen delivery. In patients who have severely restrictive or absent atrial septal defect, supplemental oxygen and other medical therapies are ineffective in treating the severe arterial desaturation, prompting emergency intervention with balloon atrial septostomy, atrial septal balloon dilation and/or stent, surgical septectomy, or immediate stage 1 palliation.
oxygen administration. For these patients, controlled ventilation is instituted with supplemental oxygen, with titration based upon both arterial and regional or venous oxygen measures by NIRS, which allows the decoding of the complex interactions of fiO2 and pCO2 on pulmonary blood flow, systemic blood flow, and total oxygen delivery. In patients who have severely restrictive or absent atrial septal defect, supplemental oxygen and other medical therapies are ineffective in treating the severe arterial desaturation, prompting emergency intervention with balloon atrial septostomy, atrial septal balloon dilation and/or stent, surgical septectomy, or immediate stage 1 palliation.
Vasoactive Medications
The need for preoperative inotropic support is variable and directed by clinical presentation and echocardiographic features. More recently, we have used noninvasive but continuous monitoring with NIRS to facilitate a less-invasive preoperative stabilization strategy (202). Patients who present in cardiogenic shock most commonly benefit from inotropic support as do patients with significantly reduced right ventricular function. On whole, inotropic catecholamines have been shown to reduce or have little effect on Qp/Qs in an animal model (195), but dose escalation may result in an undesired increase in SVR that subsequently raises Qp/Qs. For those patients in whom Qp/Qs is elevated and systemic perfusion is compromised, inodilator therapy with milrinone, a phosphodiesterase inhibitor, may be used with caution, since it may also reduce PVR and result in and undesirable increase in Qp/Qs, or excessive systemic hypotension. For the stressed neonate with increased SVR, low-dose morphine can be used to reduce agitation and work of breathing and to improve systemic oxygen balance (202) without mechanical ventilation. The neonate with progressive metabolic acidosis, or cerebral or somatic hypoxia approaching the anaerobic threshold may require mechanical ventilation, and benefit from earlier surgical intervention (229,230).
Other Adjunctive Therapies
The hypoxic patient with inefficient single-ventricle physiology benefits from increased oxygen carrying capacity. Increasing the hematocrit to 50% will benefit the patient with limited Qp or Qt. Other means to improve systemic perfusion include therapies that can attenuate sympathetic vascular tone, and low-dose dexmedetomidine may be cautiously applied. Parenteral nutrition is provided with volume administration that is consistent with gestational age, birth weight, and postnatal age. For the term infant who is greater than 2,500 g, our recommendations for fluid requirements during the first day of life are 70 to 80 cc/kg/day with escalation to 100 cc/kg/day on day of life 3, then, 120 to 140 cc/kg/day infants 4 days of age and older. The preterm infant requires more fluid due to higher transdermal insensible losses at a lower weight. Diuretic therapy is reserved for those patients who demonstrate respiratory insufficiency from increased interstitial lung edema related to either pulmonary overcirculation or restrictive pulmonary venous return.
Any of the proposed preoperative management strategies can be used in isolation or combination to balance pulmonary-to-systemic flow, optimize systemic perfusion, and preserve organ function. However, each should be embarked upon only with sufficient monitoring as they can pose potential risks to the neonate awaiting staged palliation for HLHS. Physiologically based preoperative supportive care is associated with improved outcome (125,202) including the timing of surgical intervention (231). When preoperative stabilization is inadequate to preserve extracardiac organ function particularly in neonates who present in cardiogenic shock, branch pulmonary artery banding should be considered to improved systemic perfusion and allow further organ recovery prior to traditional staged palliation (232,233).
Staged Palliation
With inadequate anatomic substrate for a two-ventricle repair, surgical approaches must address the relatively high and variable PVR in the neonate as well as the subsequent reduction in PVR that allows an eventual more stable and tolerable in-series circulation. Recognition of such physiologic necessities drove the development of numerous surgical approaches (234,235). A staged surgical pathway that was successfully championed by Norwood et al. (236,237) is now widely used. The staged approach ultimately leads the patient on a pathway to a single-ventricle in-series circulation, usually culminating in a Fontan operation with the final result similar to patients with tricuspid atresia and hypoplastic right heart syndrome (238,239). Most commonly, stage 1 palliation consists of reconstruction of the aortic arch into the right ventricular outflow, separation of branch pulmonary arteries from the right ventricle, and creation of a restrictive source of pulmonary blood flow from a systemic artery or directly from the single ventricle (237,240). Stage 2 palliation unloads the single ventricle by replacing the systemic-to-pulmonary shunt with a superior cavopulmonary anastomosis (241). The staged pathway is completed by modifications of a Fontan connection from the inferior vena cava (IVC) to the pulmonary arteries, with or without fenestration (237,239,242).
Stage 1 Palliation
Surgical Approaches
The goals of stage 1 palliation include relief of ductal-dependent systemic flow, provision of unrestricted coronary artery flow, creation of a nonrestrictive atrial septal defect to prevent pulmonary venous hypertension, and provision of a reliable but restricted source of pulmonary blood flow (Fig. 46.15). Surgical strategies are varied, and the introduction of the hybrid procedure revisits early strategies of Litwin and Van Praagh to accomplish the goals of stage 1 palliation without the use of cardiopulmonary bypass (CPB) (243,244,245,246).
Stage 1 palliation using either a modified Blalock–Thomas–Taussig shunt or right ventricle-to-pulmonary artery conduit (Sano modification) is accomplished using CPB, deep hypothermia, and altered perfusion–either circulatory arrest or regional perfusion. A connection is created between the smaller ascending aorta and the pulmonary root for provision of coronary blood flow. Restructuring of the heart’s outflow via the pulmonary root is accomplished along with relief of coarctation and arch hypoplasia. Variations in surgical techniques include resection of ductal tissue or coarctectomy as opposed to patching of the region of ductal insertion. The aim of stage 1 palliation is to create a stable anatomy that permits growth and maturation of the pulmonary vasculature so that it can accommodate subsequent single-ventricle palliation. It is important that successful surgical strategies have a low incidence of recurrent or residual lesions because these are a source of interstage mortality and can limit suitability for subsequent stages of single-ventricle palliation. Development of a restrictive atrial septal defect rarely complicates the interstage course (247). The observation that smaller ascending aortic size and the presence of aortic atresia are risk factors for mortality is an indication that coronary insufficiency is a cause of death following stage 1 palliation, and strategies that target creation of a large ascending aorta-to-pulmonary root anastomosis are likely to result in improved outcome (248,249,250,251). Arch reconstruction strategies that include coarctectomy appear to have a lower incidence of late arch obstruction (85,248).
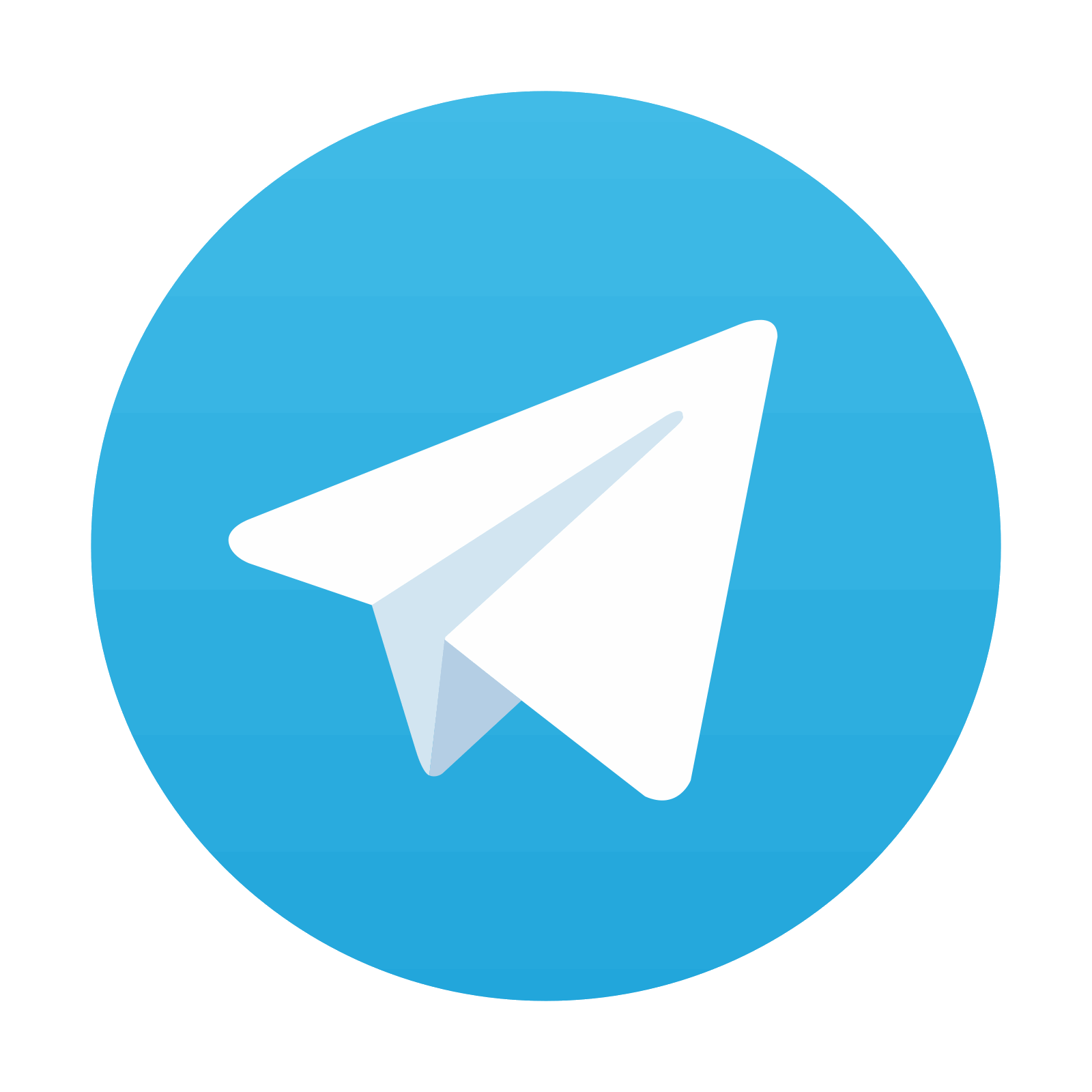
Stay updated, free articles. Join our Telegram channel
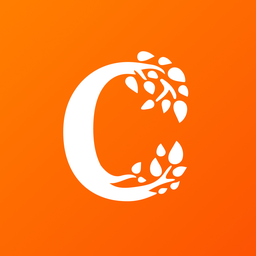
Full access? Get Clinical Tree
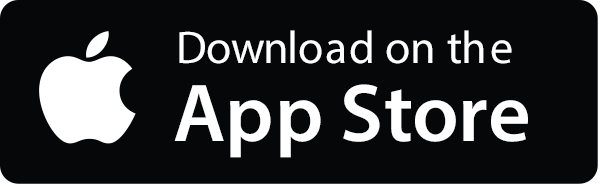
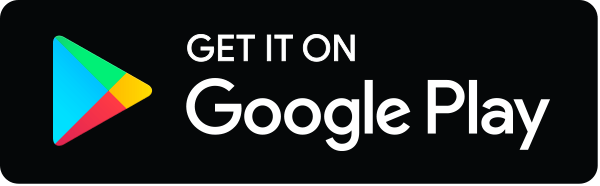
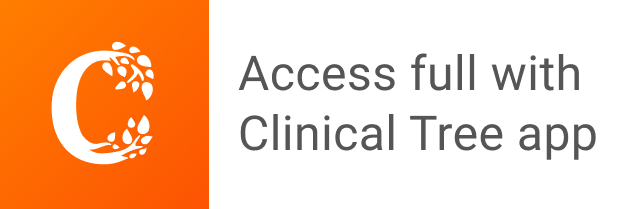