Excessive hypoxic pulmonary vasoconstriction and pulmonary hypertension Mechanism for increased capillary pressure Alterations in the pulmonary capillary barrier Mechanisms for exaggerated hypoxic pulmonary vasoconstriction Safety of reascent to high altitude This chapter explores the last of the main forms of acute altitude illness, high altitude pulmonary edema (HAPE). Unlike pulmonary edema seen at low altitude in patients with heart failure, where elevated left heart pressures play a key role in pathophysiology, HAPE develops not as the result of left ventricular dysfunction, but rather due to exaggerated hypoxic pulmonary vasoconstriction (HPV) and marked rises in pulmonary artery pressure following ascent. Affecting predominantly unacclimatized lowlanders ascending to altitudes >2500 m, as well as high altitude residents who travel to lower elevation and then reascend too quickly, it readily resolves with descent or supplemental oxygen but can be fatal if not recognized and treated appropriately. After reviewing the original descriptions of this important entity as well as the epidemiology and risk factors, the chapter addresses the most current understanding of HAPE pathophysiology, with a focus on the role of exaggerated HPV, as well as the key features of individuals prone to recurrence with repeated rapid ascents to high elevation. This discussion is followed by a thorough review of the clinical aspects, including symptoms and signs, diagnostic approach, imaging findings, and best practices for prevention and treatment. The chapter concludes by considering the expected outcomes and the safety of further ascent once symptoms resolve. The early climbing literature contains several accounts of climbers dying of “pneumonia,” but in retrospect, many of these fatalities may have actually been due to HAPE. An example was the death of Dr. Jacottet on Mt. Blanc in 1891, as described by Angelo Mosso in Life of Man on the High Alps (Mosso 1898). After climbing up to the Observatoire Vallot on August 28, he summited the mountain on September 1 and then became ill on his return to the hut at 4300 m. He remained there until he died the next night, after which an autopsy revealed “capillary bronchitis and lobular pneumonitis,” as well as “acute edema of the lung.” This latter finding was likely evidence of HAPE (West 1998). Despite cases like this, descriptions of HAPE remained uncommon in the Alps, because few unacclimatized people spent nights above 2500 m, as well as in the Himalayas where approach marches to the mountains were long enough for acclimatization to take place. The situation was different, however, in South America, where railways and roads were being built to altitudes up to 3000 m to 4000 m, thereby putting large numbers of people at risk. In 1913, Ravenhill would describe what he called puna of the cardiac type as a lethal form or altitude illness (Ravenhill 1913). Although he was wrong in attributing the condition to heart failure, his description of three cases fits well with HAPE. While Ravenhill’s descriptions were largely forgotten, several other reports would appear in the South American literature over the next 15 to 40 years. In 1927, for example, Harold Crane, the chief surgeon at a hospital in the mining town of Oroya (3750 m), described patients with soroche (mountain sickness) and cough productive of frothy sputum indicative of edema (Crane 1927). Subsequent to this, separate case series were reported by Leoncio Lizárraga Morla (Lizarraga 1955) and Arturo Bardáles Vega (Bardalez Vega 1955; Bardalez Vega 1957) in the 1950s that included extensive clinical histories, physical examinations, laboratory data, electrocardiograms, and chest radiographs. However, because the descriptions were published in Spanish language Peruvian journals with limited circulation outside of South America, knowledge of the disease remained limited in North America and Europe. Bardáles Vega did publish a very brief report in the Journal of the American Medical Association in 1956 (Bardalez Vega 1956) but this was largely overlooked (West 1998). The first extensive report of HAPE outside of South America was that of Hultgren and Spickard who described their clinical experiences in Peru in the Stanford Medical Bulletin in 1960 (Hultgren and Spickard 1960). In their article titled, “Medical experiences in Peru,” they described 41 cases of acute pulmonary edema in residents returning to altitude after a stay of five to 21 days at low altitude. They correctly suggested that these cases, which were more consistent with what is today referred to as reentry pulmonary edema rather than the form seen in unacclimatized lowlanders ascending to high altitude, was due to pulmonary hypertension rather than left ventricular failure. Because this publication was not widely read, the condition continued to receive little attention in the English-speaking medical literature until Houston published a landmark paper titled “Acute pulmonary edema of high altitude” later in the same year in the New England Journal of Medicine (Houston 1960). After this, an extensive literature on HAPE began to emerge including descriptions of cases in Indian soldiers rapidly transported to the India-China border in the 1960s (Singh et al. 1965) and physiologic studies including right heart catheterization from South America (Hultgren et al. 1964; Peñaloza and Sime 1969). This period marked the beginning of what has become an extensive body of literature drawn from all areas of the world, examining the clinical features, pathophysiology, and management of the disease. A more detailed description of this early history of work on HAPE can be found in West’s extensive review of this topic (West 1998). There are two entities that warrant consideration in a discussion of HAPE. The first is the form of the disease seen in unacclimatized lowlanders ascending to high altitude, which has been reported on a wide basis across all high altitude regions of the world. Another important form, referred to as “reentry HAPE,” is seen in long-term residents of high altitude who descend to low elevation for a period of time and then develop pulmonary edema upon reascent. Originally described in South America, this entity has also been commonly reported in Colorado (Scoggin et al. 1977) but only infrequently described in the Himalaya (Baniya et al. 2017; Wu 2004). While many of the original reports and case series included patients with reentry HAPE, the bulk of the attention in the current research literature, reviews, and clinical guidelines on high altitude medicine focuses on the form affecting unacclimatized lowlanders. That will also be the focus of this chapter, but, where relevant, information will be included on reentry HAPE. A recent report suggests there may be yet another form of the pulmonary edema at high altitude that warrants attention. Ebert-Santos (2017) has reported multiple cases of mountain residents who presented to a clinic at 2800 m with hypoxemia and radiographic changes consistent with HAPE despite no recent travel to higher or lower elevations. All cases of what was referred to in this report as mountain resident HAPE (MRHAPE), occurred in young children (ages 2 months to 15 years) and resolved readily with application of supplemental oxygen alone, although in a few cases up to 10 days of oxygen was necessary. Whether preceding viral infections played a key role in development of pulmonary edema was not clear and physiologic data such as echocardiographic estimates of pulmonary artery pressure were not available. Further work is needed to determine if this entity is present elsewhere and investigate the underlying pathophysiology and appropriate management. The incidence of HAPE is far lower among high altitude travelers than that reported for acute mountain sickness (Chapter 20). Menon (1965), for example, noted an incidence of 0.57% in soldiers flown to 3500 m in India while Hultgren and Marticorena (1978) estimated an incidence of 0.6% among adults traveling to 3750 m in Peru. Hackett et al. (1976) reported an incidence of 2.5% among trekkers in the Everest region of Nepal, where travel generally occurs between elevations of 2900 m and 5300 m, while Hultgren et al. (1996) estimated an incidence of between 0.01 and 0.1% among a general tourist population visiting a ski resort at 2900 m in Colorado. At a given elevation, however, the incidence varies markedly based on the rate of ascent. For example, the incidence of HAPE at 5500 m rises from 2.5% in those who trek to that elevation over four to six days to 15.5% in those who arrive by airlift (Singh and Roy 1969). Similarly, among a general mountaineering population, the incidence of HAPE at 4559 m is <0.2% when the ascent is undertaken over two to four days versus 10% in those with no prior history of HAPE who ascend within 22 hours (Bartsch et al. 2002). Among those with a history of HAPE, that rate may be as high as 66% (Vock et al. 1989). Less information is available regarding the incidence of reentry edema. Hultgren (1970) found the incidence among Peruvian natives varied by age with an estimated incidence of 6.4 per 100 exposures in individuals <20 years old and 0.4 per 100 exposures in those older than 21. The incidence of reentry HAPE in North America and elsewhere is unclear. HAPE typically develops two to five days following ascent to high altitude. For example, in their report of 150 cases of HAPE at a Colorado ski resort, Hultgren et al. (1996) found a mean time from arrival to symptom onset of 3 ± 1.3 days. Similarly, Kobayahsi et al. (1987) noted onset of HAPE 48–96 hours after initiation of ascent in their series of 27 consecutive patients with HAPE in Japan. Onset can occur outside of this time frame, however, as demonstrated by the fact that the time to onset in the series by Hultgren et al. (1996) ranged from one to nine days, while Singh et al. (1965) noted onset up to 10 days following arrival at high altitude in their report of an Indian soldier with HAPE. HAPE can occur at altitudes as low as 2500 m but more typically occurs above 3000–3500 m. A single study has documented a series of 52 HAPE cases over nine years at altitudes between 1400 m and 2400 m in the French Alps (Gabry et al. 2003), but this remains the only report of HAPE in otherwise healthy individuals at such elevations. In another series of 56 cases of patients evacuated from the Alps for HAPE, for example, no cases occurred below 2500 m (Hochstrasser et al. 1986). In considering the cases reported below 3000 m, it is important to recognize that in many, but not all cases, the individual diagnosed with HAPE had engaged in exercise at much higher altitudes earlier in the day. Another situation in which HAPE has been reported at much lower elevations than 2500 m is patients with underlying congenital cardiopulmonary abnormalities, such as unilateral absence of a pulmonary artery or other forms of congenital heart disease, as described further below, and again in Chapter 25 (Durmowicz 2001; Rios et al. 1985). Aside from individual susceptibility, an important issue described in further detail below, the primary risk factor for HAPE is an overly rapid ascent to high elevation, as evidenced by the changes in disease incidence as a function of ascent rate noted previously. Following the early report from Houston (1960), it has long been suspected and since demonstrated (Rashid et al. 2005) that heavy exercise following rapid ascent further increases risk, as this causes greater increases in pulmonary artery pressure, which, as noted in the pathophysiology discussion in the next section, is the key factor driving edema formation. In addition to these important variables, several other factors may also affect the risk of HAPE. Alleman et al. (2006), for example, have suggested that patent foramen ovale (PFO), a congenital condition present in approximately 25% of the general population (Hagen et al. 1984), may predispose to HAPE. In a case control study of 16 HAPE-susceptible and 19 HAPE-resistant individuals, they found that PFO was four times more common in the HAPE-susceptible than HAPE-resistant subjects and that those individuals with a PFO had significantly lower oxygen saturation following ascent than those lacking a PFO. The true risk associated with PFO is unclear, however, as the authors were unable to establish whether the PFO caused HAPE or is simply a sequelae of marked rises in pulmonary artery pressure with hypoxia and/or exercise that are the hallmark of HAPE-susceptible individuals. The fact that the incidence of HAPE is relatively low compared to the high prevalence of PFO in the general population would suggest the risk associated with PFO is not particularly high. It is also possible that, similar to the role of atrial septostomy in patients with severe pulmonary hypertension, PFO affords protection during exposure to hypoxia by providing a way to divert blood flow from the lungs and mitigate the increase in pulmonary artery pressure. Underlying pulmonary hypertension may also represent a risk factor for disease, as multiple case reports have documented development of HAPE in patients with pulmonary hypertension due to a variety of factors including unilateral absence of a pulmonary artery (Hackett et al. 1980; Rios et al. 1985), congenital heart disease (Durmowicz 2001), granulomatous mediastinitis (Torrington 1989), sarcoidosis (Brill et al. 2012), anorexigen use (Naeije et al. 1996), and portal hypertension (Bogaard et al. 2007) (Chapter 25). No cases have been described in patients with idiopathic pulmonary arterial hypertension. Because the evidence of this particular risk comes largely from case reports, it remains unclear exactly how high pulmonary artery pressure must be at baseline to increase risk or the altitudes to which an individual must ascend before their risk increases. Of the cases associated with underlying pulmonary hypertension, the most extensively described are patients with unilateral absence of a pulmonary artery. HAPE has been seen in such cases at altitudes as low as 1500 m (Rios et al. 1985) and likely develops in these cases because the pulmonary vasculature is already maximally dilated and recruited at baseline. As a result, increases in pulmonary blood flow following ascent, particularly with exertion, lead to marked rises in pulmonary artery pressure that predispose to edema formation. Several reports have also suggested that preceding viral infections may increase the risk in children (Durmowicz et al. 1997) and adults (Jacquet and Cushing 2013; Rashid et al. 2005). The precise mechanism by which such infections set the stage for pulmonary edema is not known, but may be related to the effects of inflammation on the permeability of the alveolar-capillary barrier such that it leaks with smaller increases in microvascular pressure (Durmowicz et al. 1997). In general, pulmonary edema develops because of an imbalance in the Starling forces and, in particular, either an increase in hydrostatic pressure or an increase in capillary permeability. Changes in oncotic pressure may play less of a role in the lungs as a result of unique properties of the interstitium, lymphatics (Miserocchi 2009), and pulmonary vascular endothelium (Parker 2007). An extensive body of research over many years has established that HAPE primarily results from an increase in pulmonary capillary hydrostatic pressure, as well as an increase in pulmonary capillary permeability due to excessive hypoxic pulmonary vasoconstriction and a significant increase in pulmonary artery pressure following ascent. These and other mechanisms are considered in detail in several extensive reviews on this topic (Bartsch et al. 2005; Swenson and Bartsch 2012) while the key issues are highlighted below. The presence of markedly increased pulmonary artery pressure was initially recognized in many of the early studies of HAPE in the 1960s during which patients underwent right-heart catheterization and direct measurement of pulmonary artery pressure prior to initiation of treatment (Hultgren et al. 1964; Peñaloza and Sime 1969). More recently, this phenomenon was nicely demonstrated by Maggiorini et al. (2001), who performed right-heart catheterization in both HAPE-susceptible and non-HAPE-susceptible individuals following rapid ascent to 4559 m and found that mean pulmonary artery pressure, as well as pulmonary capillary pressure, was higher among the HAPE-susceptible individuals who developed HAPE on that particular ascent than among the healthy controls and the HAPE-susceptibles who remained healthy (Figure 22.1). The link between the rise in pulmonary artery and pulmonary capillary pressures was nicely established by Swenson et al. (2002), who performed bronchoalveolar lavage in both HAPE-susceptible and non-HAPE-susceptible individuals following rapid ascent to 4559 m and demonstrated dose-dependent increases in red blood cell counts and serum-derived protein concentrations in the lavage fluids of subjects with HAPE and in those who developed HAPE within the subsequent 24 hours; the higher the pressure, the greater the number of red cells and concentration of proteins in the lavage fluid (Figure 22.2). Figure 22.1Mean pulmonary artery pressure (Ppa) and pulmonary capillary pressure (Pcap) in 14 healthy controls (△) and 16 HAPE-susceptible individuals at 4559 m. (⚬) denote HAPE-susceptible individuals who did not develop HAPE during the study. (⦁) denote those HAPE-susceptible individuals who developed HAPE. The horizontal bars show the mean values. *P <0.05. **P <0.01 vs. controls. †P <0.01 vs. non-HAPE. (Source: Maggiorini et al. 2001.) Figure 22.2Individual bronchoalveolar lavage (BAL) red blood cell count (A) and albumin concentrations (B) versus estimated pulmonary artery (PA) systolic pressures at 4559 m. (⚬) represent normal values at 490 m. (⦁) represent HAPE-resistant subjects. (◾) represent HAPE-susceptible individuals who did not develop HAPE during the study. (▴) represent HAPE-susceptible individuals who developed HAPE during the study. The vertical lines denote the threshold systolic PA pressure above which red blood cells (A) and albumin (B) appear in BAL fluid. (Source: Swenson et al. 2002.) It is well established that increased capillary pressure in HAPE is not related to left ventricular dysfunction as multiple right-heart catheterization studies have demonstrated normal pulmonary capillary occlusion pressure, indicative of normal left heart pressures and function (Hultgren et al. 1964; Maggiorini et al. 2001; Peñaloza and Sime 1969). Instead, the primary factor driving the increase in capillary pressure is increased inflow from the arterial side of the pulmonary circulation. The major question that arises, however, is how such an increase in flow can be seen following pulmonary arterial vasoconstriction, as pulmonary edema is not generally seen in other states of high pulmonary arterial resistance such as pulmonary arterial hypertension. In the case of HAPE, the key factor appears to be that hypoxic pulmonary vasoconstriction is uneven. Those areas in which vasoconstriction occurs experience a decrease in flow while unaffected areas see an increase in flow due to the increase in upstream pulmonary artery pressure which, in turn, raises downstream capillary hydrostatic pressure This concept of uneven vasoconstriction, which would account for the patchy distribution of opacities often seen on radiographic imaging in patients with HAPE, was initially suggested by Hultgren, who showed that progressively tying off increasing portions of the pulmonary arterial tree in a dog, thereby forcing the total cardiac output through only a portion of the lung, led to edema formation in the perfused segments of lung (Hultgren 1969; Hultgren et al. 1966). Subsequent studies have provided further support for this concept. Viswanathan et al. (1979), for example, performed lung perfusion scans in 12 individuals with a prior history of HAPE before and after breathing an FIO2 of 0.10 and noted a marked increase in perfusion in localized areas as well as significantly more perfusion heterogeneity than seen in controls with no prior history of HAPE. This finding has been replicated in more recent studies using MRI (Dehnert et al. 2006; Hopkins et al. 2005). Hopkins et al. (2005) studied pulmonary blood flow in hypoxia in HAPE-susceptible and HAPE-resistant individuals as well as unselected individuals with no prior high altitude exposure. Following exposure to normobaric hypoxia (an FIO2 of 0.12), during which time there were no differences in oxygen saturation between the three groups, the relative dispersion of signal intensity, a marker of perfusion heterogeneity, was increased in the HAPE-susceptible individuals but not in the other two groups, suggesting the HAPE-susceptible individuals experienced uneven hypoxic pulmonary vasoconstriction. In a subsequent MRI study, Patz et al. (2017) found decreased ventilation heterogeneity in HAPE-susceptible individuals compared to HAPE-resistant controls, suggesting that uneven HPV is caused by vascular abnormalities rather than differences in ventilation. In addition to uneven HPV and the subsequent overperfusion of unprotected regions of the vascular tree, another factor may contribute to the increase in pulmonary capillary pressure is hypoxic pulmonary venoconstriction. Studies have demonstrated, for example, that the pulmonary veins constrict in response to hypoxia (Raj and Chen 1986; Zhao et al. 1993) and that venous constriction may account for up to 20% of the total increase in pulmonary vascular resistance with hypoxic exposure (Audi et al. 1991). Such venoconstriction can limit outflow from the capillary bed, thereby further increasing capillary pressure, particularly in over perfused areas. While an increase in capillary hydrostatic pressure, in and of itself, can cause pulmonary edema through alterations in Starling forces, as happens in left heart failure, changes in the pulmonary capillary permeability may also play a role in the development of HAPE. Suspicion for such changes was raised by bronchoalveolar lavage studies demonstrating the presence of high molecular weight proteins and cells in the alveolar spaces of patients with HAPE (Schoene et al. 1988). West and Mathieu-Costello (1992) and West et al. (1991) have proposed that this observation and the development of HAPE can be accounted for by stress failure of the pulmonary capillaries due to high wall stresses associated with the increase in capillary transmural pressure. This hypothesis was based on an extensive body of research demonstrating that increases in capillary transmural pressure cause ultrastructural damage to the capillary wall including disruption of the endothelial layer, the alveolar epithelial layer, and, in some cases, the entire barrier (Costello et al. 1992; Elliott et al. 1992; Tsukimoto et al. 1991; West et al. 1991). West et al. (1993), for example, demonstrated in a rabbit model that increasing the transvascular pressure to 40–60 cm H2O over one minute caused rupture of the basement membrane and the alveolar-capillary barrier. Figure 22.3 provides an example of this phenomenon, demonstrating movement of a red cell across the capillary wall of a rat pulmonary capillary exposed to a barometric pressure of 294 mmHg for four hours (West et al. 1995). The duration of exposure in this study, however, is far shorter than the time frame over which HAPE typically develops in humans. Figure 22.3Electron micrograph of a pulmonary capillary (c) in a rat exposed to a barometric pressure of 294 mmHg for four hours. The wall of the vessel has ruptured and a red blood cell is moving through the defect in the wall (arrows) from the capillary to the alveolar space (a). (Source: West et al. 1995.) Swenson and Bartsch (2012) have cautioned against broad application of the term “stress failure” to account for all cases of HAPE, arguing that in individuals who remain at rest or engage in mild to moderate exercise, the increase in capillary pressure may not be sufficient to cause overt structural failure of the alveolar-capillary barrier. Instead, they argue that early or milder forms of the disease may be related to nontraumatic processes marked by dynamic, pressure-sensitive changes in transcellular vesicular transport or the size of pores and fenestrations (Bartsch et al. 2005). This hypothesis could account for the rapid resolution of symptoms and signs in many HAPE patients following descent, a time course that would not necessarily be expected in cases of overt structural failure of the alveolar-capillary barrier. In the end, it is likely that changes in the permeability of the alveolar capillary barrier occur across a spectrum in which the more dynamic processes noted above play a greater role in milder forms of illness and overt structural failure is present only in the most severe cases (Swenson and Bartsch 2012). As noted above, a key factor in the development of HAPE is the excessive pulmonary vascular response to hypoxia and the subsequent large increase in pulmonary artery pressure. This exaggerated response is likely the result of multiple factors. Enhanced hypoxic pulmonary vasoconstriction may result from reduced availability of the potent vasodilator, nitric oxide (NO), and its second messenger cGMP. HAPE-susceptible individuals, for example, have been shown to have decreased exhaled NO concentrations during acute hypoxic exposure at low elevation and during more prolonged exposure at terrestrial high altitude compared to nonsusceptible individuals (Figure 22.4) (Busch et al. 2001; Duplain et al. 2000), while mountaineers with HAPE have lower concentrations of nitrate and nitrite in bronchoalveolar lavage fluid compared to healthy controls (Swenson et al. 2002). While these studies do not allow one to determine whether these differences in NO availability are related to differences in production by the vascular endothelium versus the alveolar and bronchial epithelium, Berger et al. (2005) studied changes in the systemic circulation as a reasonable surrogate for events in the pulmonary circulation. They demonstrated that following 90 minutes of hypoxic exposure, HAPE-susceptible individuals have lower plasma nitrite concentrations compared to nonsusceptible individuals as well as less endothelial-dependent vasodilation in response to acetylcholine administration. These results suggest that differences in the vascular endothelium accounts for at least part of the reduced NO availability. While these studies strongly point to a role for NO bioavailability in the development of HAPE, administration of inhaled NO to individuals with HAPE does not normalize pulmonary artery systolic pressure (PASP) (Anand et al. 1998), suggesting that other factors are playing a role in the exaggerated pulmonary vascular response. Figure 22.4Left: exhaled nitric oxide (NO) after 40 hours at 4559 m in individuals developing HAPE and in individuals not developing HAPE (HAPE-R) despite identical exposure to high altitude (Duplain et al. 2000). Right: exhaled NO in HAPE-susceptible (HAPE-S) and non-HAPE-susceptible (HAPE-R) individuals after 4 hours of exposure to hypoxia (FIO2 = 0.12) at 100 m. N, number of subjects. (Source: Busch et al. 2001.) Endothelin-1 (ET-1) is another vasoactive mediator with pulmonary vascular effects, but its role in HAPE remains unclear. Differences in circulating levels between HAPE-susceptible and nonsusceptible individuals are not apparent with acute exposures to hypoxia (<90 minutes) (Berger et al. 2005) during which time HPV has already commenced, but they do manifest after one to two days at high altitude (Sartori et al. 1999b). A transpulmonary gain in ET-1 concentration has also been noted when assessed after one to two days at 4559 m and is associated with higher PASP at high altitude (Berger et al. 2009). Together, these results suggest that rather than affecting the initial pulmonary vascular responses to hypoxia, ET-1 may play a role as the individual spends more time at high altitude. The role of circulating catecholamines also remains unclear. Duplain et al. (1999) have shown that HAPE-susceptible individuals have increased sympathetic activity, as assessed by postganglionic nerve discharge in skeletal muscle compared to healthy controls. Similarly, other studies have shown that sympathetic stimulation via alpha receptors augments HPV (Brimioulle et al. 1997; Ming and Wang 1989), and administration of the alpha antagonist, phentolamine, reduces pulmonary vascular resistance and pulmonary artery pressure in individuals with HAPE and healthy controls at high altitude (Hackett et al. 1992). On the other hand, Berger et al. (2011) found no differences in transpulmonary epinephrine or norepinephrine concentrations at rest after 20 hours at 4559 m and no correlation between the transpulmonary concentration changes and PASP. Beyond these mediators, other factors including free radicals (Bailey et al. 2010), reactive oxygen species (Waypa et al. 2010), hydrogen sulfide (Prieto-Lloret and Aaronson 2017), angiotensin II (Kleinsasser et al. 2012), and arachidonic acid metabolites (Han et al. 2013) may affect HPV and subsequent changes in PASP following ascent; however, aside from a study by Bailey et al. (2010) in which they found transpulmonary increases in free radical concentrations that were more pronounced in three subjects with HAPE, no studies have examined the link between these mediators and the risk of HAPE. Several studies have demonstrated that HAPE-susceptible individuals have blunted hypoxic ventilatory responses (HVR) (Hackett et al. 1988; Matsuzawa et al. 1989; Schirlo et al. 2002), which could affect the pulmonary vascular response to hypoxia by one of several means. In addition to lowering PAO2 at a given elevation and providing a stronger stimulus for HPV, a blunted ventilatory response will cause smaller changes in PaCO2, thereby alleviating some of the effects of hypocapnia on HPV (Balanos et al. 2003). Blunted HVR may also affect HPV via its effect on the PaO2, as it has been shown that perfusion of the bronchial circulation with deoxygenated blood leads to an increase in pulmonary vascular resistance (Marshall et al. 1991). Finally, there is evidence from both animal models and humans of a direct effect of the peripheral chemoreceptors on the magnitude of HPV that is independent of the changes in PAO2. For example, Wilson and Levitzy (1989) demonstrated that when minute ventilation is fixed in anesthetized dogs, carotid body stimulation reduces HPV, while Albert and Swenson (2014) measured normobaric poikilocapnic HVR and PASP in healthy humans and demonstrated an inverse correlation between HVR and HPV. Several studies have reported differences in baseline lung volumes in HAPE-susceptible and nonsusceptible individuals, which may account for the differences in pulmonary vascular responses. Viswanathan et al. (1969), for example, reported reduced forced vital capacity (FVC) and total lung capacity (TLC) in Indian soldiers with a history of HAPE, while Steinacker et al. (1998) found smaller functional residual capacity (FRC) as well as FVC and TLC in a group of HAPE-susceptible individuals when compared to healthy controls. Smaller lung volumes may be associated with a reduced pulmonary vascular bed and, therefore, decreased ability to recruit pulmonary vessels as blood flow increases in hypoxia, particularly with exercise. The net result would be larger increases in pulmonary artery pressure in hypoxia than would occur in those with higher lung volumes and a larger pulmonary vascular bed. This proposed mechanism has never been firmly established but is supported somewhat by evidence that the diffusion capacity for carbon monoxide, a marker of the surface area for gas exchange, is lower in hypoxia and with exercise and increases less with hypoxic exercise in HAPE-susceptible compared to non-HAPE-susceptible individuals (Steinacker et al. 1998). In addition to increased pulmonary vascular reactivity, several other factors have received attention in the literature for their potential role in the development of HAPE. While the interactions between hydrostatic pressure and capillary permeability are the primary factors affecting alveolar fluid balance, alterations in sodium and water transport via apical epithelial Na+ channels (ENaC) and basolateral membrane Na+/K+-ATPases may also contribute to edema formation. Various studies in rats and mice, for example, have demonstrated that hypoxia decreases expression and activity of the apical ENaC and basolateral Na+/K+-ATPases through alterations in gene transcription and impaired β2-adrenergic receptor signaling (Baloglu et al. 2009; Planes et al. 1997; Zheng et al. 2009), decreases transepithelial alveolar sodium transport (Mairbaurl et al. 2002), and decreases alveolar fluid clearance (Vivona et al. 2001). Similarly, ENaC-deficient mice accumulate more alveolar fluid in hypoxia relative to ENaC-replete mice (Egli et al. 2004). Because it is difficult to study the lung alveolar epithelium in humans, the possible link between HAPE and alterations in sodium and water transport has been investigated by examining the nasal epithelium as a surrogate marker of lung alveolar epithelial function. As with the alveolar epithelium, nasal epithelial sodium transport is inhibited by hypoxia (Mairbaurl et al. 2003b), and several studies have shown that HAPE-susceptible individuals have reduced nasal potential differences suggestive of decreased ENaC-mediated sodium reabsorption and, therefore, reduced sodium and water transport across the respiratory epithelium (Sartori et al. 2004). Other studies, however, have suggested the observed changes are related to differences in chloride secretion via the cystic fibrosis transmembrane regulator rather than alterations in ENaC activity (Mairbaurl et al. 2003a). Further support for the role of alterations in sodium transport in development of HAPE comes from clinical studies in which use of the β2-receptor agonist, salmeterol, and the glucocorticoid, dexamethasone, two agents capable of upregulating ENaC and Na+/K+-ATPases and blunting their hypoxia-mediated down regulation, decreased the incidence of HAPE in known susceptible individuals (Maggiorini et al. 2006; Sartori et al. 2002). Despite these positive results, it has been difficult to establish that salmeterol and dexamethasone exerted these effects specifically through alterations in sodium and water transport. β agonists, for example, have also been shown to also blunt HPV, increase HVR, and upregulate production of the vasodilator nitric oxide and tighten cell junctions (Adding et al. 1999; Ballester et al. 1989; Easton et al. 2008; Reyes et al. 1978; Unwalla et al. 2012), while dexamethasone has also been shown to significantly blunt increases in pulmonary artery pressure seen with exercise in hypoxia (Fischler et al. 2009). Each of these factors could, in and of themselves, have a protective effect against HAPE. Until further studies can be done with agents that selectively affect ENaC and Na+/K+-ATPase function in the alveolar epithelium, the precise contribution of alterations in sodium and water clearance to development of HAPE will remain unclear (Swenson and Bartsch 2012). Bronchoscopy with bronchoalveolar lavage performed on individuals who developed HAPE while climbing Denali demonstrated increased cellularity and increased concentrations of inflammatory mediators such as leukotriene B4 and thromboxane B2 (Schoene et al. 1986; Schoene et al. 1988), suggesting that inflammatory responses might play a role in increasing capillary permeability beyond the mechanical factors noted above. Later studies performed on patients with HAPE in Japan demonstrated similar results (Kubo et al. 1998; Kubo et al. 1996). These observations could explain the observed association between preceding viral upper respiratory tract infections and HAPE in children (Durmowicz et al. 1997). One challenge with these bronchoalveolar lavage studies, however, was that bronchoscopy was performed at varying times relative to the development of HAPE, which made it difficult to determine whether inflammatory responses were present early and, therefore, contributed to edema formation or were a late phenomenon in response to the already established edema. This question was addressed by Swenson et al. (2002) who performed bronchoscopy with bronchoalveolar lavage in HAPE-susceptible individuals and healthy controls within the first 24 hours of arrival at 4559 m and at the first signs of HAPE. While the lavage fluid of the individuals with HAPE demonstrated increased protein concentration and red blood cell counts that correlated with the increase in pulmonary artery pressure, there was no increase in the number of leukocytes, proinflammatory cytokines, and eicosanoids. These results are in agreement with those of Kleger et al. (1996) who examined individuals who developed HAPE at 4559 m and found that plasma concentrations of fibrinogen, α1-acid glycoprotein, C-reactive protein, and IL-6 were unchanged compared to low elevation in the early period following arrival at 4559 m and only increased significantly toward the end of the 40-hour stay at that elevation. TNF-α and F2-isoprostane levels did not change at any point following arrival. Together, these studies suggest that the timing at which one looks for evidence of inflammation or other factors contributing to the development of edema is key for understanding the pathophysiology of HAPE and, importantly, provide strong evidence that mechanical factors, rather than underlying inflammation, are the primary drivers of edema formation. Pulmonary artery pressure rises in hypoxia as result of hypoxic pulmonary vasoconstriction as well as increases in pulmonary blood flow, with the latter affected by a variety of factors including the compliance of the pulmonary vasculature. To address this issue further, Mulchrone et al. (2019) expanded an analysis of previously published data (Eldridge et al. 1996) and measured changes in pulmonary arterial stiffness with exercise, acute hypoxia, and hypobaria in seven HAPE-susceptible and nine nonsusceptible controls at sea level and again at 3810 m and found that the HAPE-susceptible individuals had increased proximal and distal pulmonary arterial stiffening with exercise and hypoxia compared to the controls. This decreased compliance could limit the ability to recruit and distend pulmonary vessels as cardiac output increases, leading to greater rises in pulmonary artery pressure, which contributes to edema formation by the mechanisms described above, although, as the authors noted, the differences in stiffness could reflect the sequelae of prior episodes of HAPE rather than a cause of the problem. This work validates earlier data from Steinacker et al. (1998), which demonstrated that the diffusion capacity for carbon monoxide in hypoxia and with exercise was lower in HAPE-susceptible individuals than nonsusceptible controls. This finding was attributed to differences in vascular capacitance, which could be secondary to differences in pulmonary arterial stiffness or differences in lung volume affecting the cross-sectional area for blood flow. Differences in pulmonary vascular responses, alveolar fluid clearance, inflammatory responses, and other factors that may contribute to the development of HAPE are likely related to genetic polymorphisms that affect the physiologic responses to hypoxia in a given individual. HAPE susceptibility varies among populations and clusters within families, providing support for a genetic basis (Luo et al. 2012). In the past 20 years, interest has increased in identifying such markers as a way to predict susceptibility and enhance understanding of disease pathophysiology. Given the observed differences in NO production in HAPE-susceptible individuals, attention has been focused on polymorphisms in genes coding for endothelial-derived NO synthase (e-NOS). Several studies have noted an association between eNOS gene polymorphisms and HAPE in patients of Japanese (Droma et al. 2002) and Indian (Ahsan et al. 2004) origin, but this association was not borne out in a study of Caucasian individuals (Weiss et al. 2003). Positive associations have also been reported for a variety of other genes including those encoding for bone-morphogenic protein-2, activin-receptor-like kinases, the serotonin transporter, and serotonin (Ali et al. 2016) as well as apolipoprotein A-1 and haptoglobin (Ahmad et al. 2011), while other studies have failed to establish a relationship between HAPE and ACE I/D gene polymorphisms (Dehnert et al. 2002) or polymorphisms in genes encoding for VEGF (Hanaoka et al. 2009). More recent work has shown that there are distinct molecular signatures during the acute and recovery phases of HAPE, reflecting the downstream effects of HIF-1α on hypoxia-related genes such as BNIP3L, VEGFA, ANGPTL4, and EGLN1, that differ from those seen in healthy controls (Sharma et al. 2014; Yuhong et al. 2018). Overall, while several intriguing associations have been reported, the best that can be said at this point is that multiple polymorphisms, rather than a single variant, may affect susceptibility to HAPE. Further work will be required to sort out the specific polymorphisms that are playing a role, the interactions between them, and the downstream consequences. While there are many idiosyncratic cases of HAPE in which affected individuals never have problems on subsequent ascent to high altitude, there is a cohort of individuals who are prone to recurrence with repeated ascent to the roughly the same elevation at approximately the same rate. Such individuals have served as the patient population for a large number of studies of the pathophysiology, prevention, and treatment of HAPE (Bartsch et al. 1989; Bartsch et al. 1991; Maggiorini et al. 2006; Maggiorini et al. 2001; Sartori et al. 2002; Swenson et al. 2002). Based on studies in the Alps, it has been estimated that they have a roughly 60% risk of recurrence with repeat travel to high altitude (Vock et al. 1989). Throughout the literature on HAPE, these individuals are often referred to as “HAPE-susceptible,” a term used throughout this chapter, in fact. This term is somewhat problematic, however, as it implies there is a cohort of people who are resistant to HAPE, when, in fact, most people can develop HAPE if they ascend at an overly rapid rate to too high an elevation, particularly when engaging in heavy exercise. Similarly, people with a history of repeated episodes of HAPE have later ascended to very high elevations by slowing their ascent rate to an adequate level (Bartsch 1999). As a result, individuals cannot be viewed as susceptible or resistant to HAPE under all circumstances and, instead, should only be considered as such when taking into account the altitude to which they are ascending and the rate at which they travel there. This issue may not be critical from a clinical standpoint, as any history of HAPE likely warrants slow ascent and pharmacologic prophylaxis with future mountain travel, but it is very important when considering research studies designed to investigate disease pathophysiology and the features of people prone to recurrence. An individual who developed HAPE when ascending to 6000 m at a rate of 600 m/day, for example, may not develop HAPE when in a study in which climbers only ascend to 4500 m at a rate of 400 m/day. As a result, they may not manifest pathophysiologic responses of interest in a particular study. Similarly, in a study on pharmacologic prophylaxis, the absence of HAPE in the latter situation might be attributed to an effect of the medication when, instead, it may have been related to the slower ascent. For these reasons, studies should be focused on individuals who have developed HAPE in a setting similar to that used in the intended study. This approach is a feature of many of the high quality studies examining aspects of HAPE pathophysiology and prevention from the Alps (Bartsch et al. 1989; Bartsch et al. 1991; Maggiorini et al. 2006; Maggiorini et al. 2001; Sartori et al. 2002; Swenson et al. 2002) as they included subjects who had at least two episodes of HAPE with rapid ascents to around 4500 m, the same ascent approach used in the research studies. The most noteworthy feature of individuals appropriately classified as HAPE-susceptible is their exaggerated pulmonary vascular response to hypoxia, which, as noted above, is the key pathophysiological factor in the development of HAPE. This was nicely demonstrated in a study by Grunig et al. (2000) in which they estimated pulmonary artery systolic pressure at rest in hypoxia (FIO2 = 0.12) and during exercise in normoxia in both HAPE-susceptible and control subjects and found significantly higher pressures in the HAPE-susceptible group. Similarly, Dehnert et al. (2005) estimated pulmonary artery systolic pressure at rest in hypoxia and with exercise in both normoxia and hypoxia in similar groups of subjects and found significantly higher values under each condition in the HAPE-susceptible group when compared to controls (Figure 22.5). The mechanisms that account for the exaggerated hyper-responsiveness have not been clearly identified. Figure 22.5Individual estimated pulmonary artery systolic pressure (PASP) values for HAPE-resistant controls (A) and HAPE-susceptible individuals (B) at baseline, after two hours of normobaric hypoxia (FIO2 0.12), and during exercise in normobaric hypoxia (FIO2 0.12). Exercise values represent the maximum value achieved under that condition for each individual. The solid line represents the optimal cut off for distinguishing HAPE-susceptible individuals from controls during exercise in hypoxia, while the dotted line is the optimal cut off for rest in hypoxia. (Source: Dehnert et al. 2005.) While exaggerated HPV is a common feature of HAPE-susceptible individuals, the presence of this phenotype is not sufficient to cause HAPE. Dehnert et al. (2015) screened 421 altitude-naïve individuals at low elevation for exaggerated pulmonary artery pressure responses to resting hypoxia to identify a cohort with exaggerated HPV. This cohort, along with a group of matched controls with normal HPV, ascended rapidly to 4559 m where they remained for 48 hours. Only four of the 39 individuals with exaggerated HPV subsequently developed HAPE, far lower than the reported 60% recurrence rate for HAPE noted among HAPE-susceptible individuals with similar ascent profiles to the same elevation. These results, which were similar to those from an earlier study (Sartori et al. 1999a), suggested that exaggerated HPV is a necessary but not sufficient condition for HAPE and that some other factor(s) must also play a role. Betz et al. (2015) tested the concept that differences in alveolar fluid reabsorption could be the key additional factor by measuring nasal potential differences in normoxia in high altitude tolerant controls, HAPE-susceptible individuals with high HPV, and HAPE-resistant individuals with high HPV. Although nasal potential differences were lower in the HAPE-susceptible subjects with high HPV than controls, there were no statistically significant differences between the values seen in the high HPV–HAPE-resistant group and those in either the HAPE-susceptible or control groups. This result, which could have been related to the small number of subjects in each group, argues against a clear role for differences in alveolar fluid clearance as the key differentiating factor that can explain observed differences in HAPE susceptibility among those with high HPV. No other studies have identified other factors that can explain this difference. Common symptoms of HAPE, as reported in two of the largest series of HAPE patients available, are presented in Table 22.1. In the early stages of the disease, affected individuals usually report dyspnea on exertion out of proportion to their earlier experiences at high altitude or that experienced by other individuals traveling at the same elevation. They require frequent rest breaks and increased time to recover from any exertion. Fatigue, weakness, dry cough, and a sensation of gurgling in the chest may also be present. As the disease progresses, the individual becomes dyspneic with mild exertion or at rest, while the cough worsens and, in very late stages, may become productive of pink frothy sputum. In many, but not all cases, HAPE is either preceded or accompanied by symptoms of acute mountain sickness (AMS). Menon N = 101 Symptom Number of cases Percent Dyspnea 84 83 Chest pain 66 65 Headache 63 62 Nocturnal dyspnea 59 58 Dry cough 51 50 Hemoptysis 39 39 Nausea 26 26 Insomnia 23 23 Dizziness 18 18 Hultgren et al., N = 150 Symptom Number of cases Percent Dyspnea 115 77 Cough 103 69 Headache 80 53 Chest congestion 62 41 Nausea 52 35 Fevers, chills, sweats 45 30 Weakness, fatigue 38 25 Insomnia 32 21 Dizziness, lightheadedness 25 17 Malaise 17 11 Palpitations 14 9 Sources: Menon (1965) and Hultgren et al. (1996). Vital sign abnormalities vary based on the severity of illness and include tachycardia, tachypnea, and decreased oxygen saturation (SpO2). Fever may be present but typically does not exceed 38.5°C. In addition to perioral and nail bed cyanosis, individuals have crackles on lung auscultation. These can be localized to the right middle lung fields in early disease but become more diffuse as the disease worsens. Many patients with HAPE also manifest confusion or altered mental status. This can be related to hypoxic encephalopathy or be due to concurrent HACE, which often develops in cases of prolonged and/or severe untreated HAPE. Supplemental oxygen administration can help determine the etiology of mental status changes in these cases. Resolution of encephalopathy within minutes of sufficient increases in SpO2 would point strongly to HAPE as the underlying cause, as manifestations of HACE would not resolve so quickly with oxygen administration. Worsening dyspnea should prompt consideration of other diagnoses including asthma exacerbation, myocardial infarction, pneumonia, pneumothorax, pulmonary embolism, and even anxiety. Because diagnostic tests, including chest radiography and electrocardiography, are often absent in remote field locations, sorting through the differential can be challenging. One potential tool to guide diagnosis is the Lake Louise Consensus Definition of HAPE (Table 22.2) (Hackett and Oelz 1992). While this tool has the benefit of relying on symptoms and signs for diagnosis, rather than laboratory studies and imaging, it was intended more for defining cases in research studies rather than clinical practice and may not be something about which all clinicians are aware. In addition, the criteria are nonspecific and patients with pneumonia or pulmonary embolism could satisfy the case definition. Symptoms (at least two of) Dyspnea at rest Cough Weakness or decreased exercise performance Chest tightness or congestion Signs (at least two of) Crackles or wheezing in at least one lung field Central cyanosis Tachypnea Tachycardia Source: Bartsch et al. (1993). In many cases, clinicians weigh other factors in the evaluation of dyspneic patients beyond those in the consensus definition. For example, the timing of onset can be helpful as myocardial infarction, pneumothorax, and pulmonary embolism would be expected to present in a very acute manner, whereas HAPE typically worsens over a period of hours to days. Asymmetric lower extremity edema is sometimes, but not always, present in those with pulmonary embolism. As noted, patients with HAPE are hypoxemic compared to normal individuals and those with anxiety, but this problem can be seen with many of the other entities on the differential as well. Given the diagnostic uncertainty that may persist despite a thorough history and physical exam, the prudent course for individuals with worsening dyspnea of unclear etiology is to evacuate to a lower elevation for a more thorough evaluation. Resolution of symptoms with descent would provide further evidence that the affected individual was suffering from HAPE. Plain chest radiographs typically demonstrate fluffy, cotton-wool-like opacities consistent with an alveolar-filling process. Two examples are shown in Figure 22.6. The appearance of these opacities is highly variable between individuals as well as between episodes of HAPE in individuals with recurrent disease; they can be unilateral or bilateral, central and/or peripheral, and patchy or homogenous (Vock et al. 1991; Vock et al. 1989). In many cases, they are more prominent in the right-middle to lower lung zones, but some patients show no superior-inferior difference (Vock et al. 1991). The opacities may be more prominent than suggested by the clinical exam in early HAPE (Menon 1965), progress over time as the disease worsens (Vock et al. 1989), and resolve readily as the clinical condition improves. In addition, affected individuals may demonstrate engorged pulmonary arteries and right ventricular enlargement (Koizumi et al. 1994; Marticorena et al. 1964; Vock et al. 1989), but findings supportive of a cardiogenic process, including left ventricular or left atrial enlargement, Kerley-B lines, or pleural effusions, are typically absent (Vock et al. 1989). Figure 22.6Representative examples of chest radiography findings in HAPE. (A) A 47-year-old man with HAPE at 4559 m. The opacities are asymmetric and most prominent in the right middle and lower lobes. (Image courtesy of Marco Maggiorini.) (B) A 47-year-old woman who developed HAPE in Colorado. Unlike in (A), the opacities are present in the bilateral lower lung zones. (Image courtesy of Peter Hackett.) In patients with a compatible history, exam, and plain chest radiograph, chest CT scans are generally not necessary to diagnose HAPE. Compared to the situation with plain chest radiographs, descriptions of CT findings in HAPE are limited. When performed, they may demonstrate nonspecific, asymmetric patchy, ground glass opacities often with a peribronchovascular distribution, and subpleural sparing. Interlobular septal thickening, pleural effusions, and cardiomegaly are typically lacking (Gluecker et al. 1999; Lindholm et al. 2018; Martinez-Jimenez et al. 2006). Chest ultrasonography may reveal the presence of “B-lines,” also referred to as “comet tails,” and given the decreasing size and increasing portability of these systems, ultrasonography may provide an alternative means of diagnosing HAPE in the absence of chest radiography. In a series of patients with HAPE at Pheriche in Nepal (4240 m), Fagenholz et al. (2006), for example, found higher comet-tail scores in individuals with HAPE compared to healthy controls as well as a negative correlation between the comet-tail score and oxygen saturation. More recently, Yang et al. (2018) performed lung ultrasound on 148 patients admitted to a hospital with acute respiratory problems, the majority of whom were ultimately determined to have HAPE, and noted a higher sensitivity than plain chest radiography for the diagnosis, as determined by a group of blinded observers. Because comet tails can be seen in asymptomatic individuals following ascent to high elevation (Pratali et al. 2010; Strapazzon et al.
Introduction
Historical Perspective
Clinical Entities
Incidence
Timing and Altitude of Onset
Risk Factors
Pathophysiology
Excessive hypoxic pulmonary vasoconstriction and pulmonary hypertension
Mechanism for increased capillary pressure
Alterations in the pulmonary capillary barrier
Mechanisms for exaggerated hypoxic pulmonary vasoconstriction
Circulating Vasoactive Mediators
Ventilatory Responses to Hypoxia
Lung Volumes
Other contributing factors
Alterations in Alveolar Fluid Clearance
Inflammation
Pulmonary Artery Stiffness with Exercise and Hypoxia
Genetic factors
Predisposition to Hape
Defining susceptibility to HAPE
Characteristics of individuals predisposed to HAPE
Clinical Features
Symptoms and signs
Differential diagnosis
Imaging findings
Plain Chest Radiography
Chest CT Scans
Ultrasonography
Stay updated, free articles. Join our Telegram channel
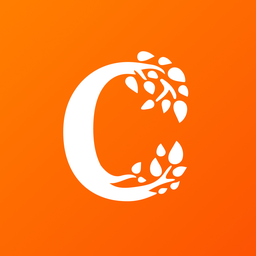
Full access? Get Clinical Tree
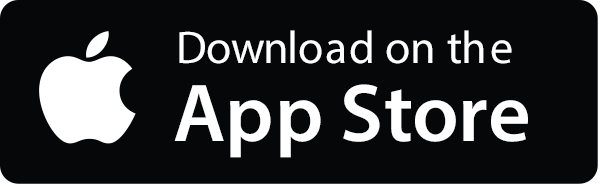
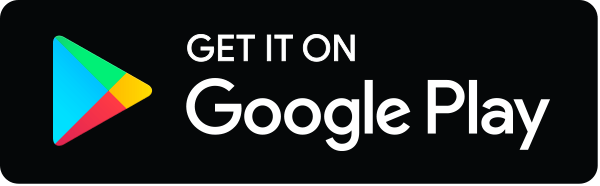