Effect of high altitude on plasma volume The effect of posture on plasma volume Effect of acute hypoxia on plasma volume Effect of chronic hypoxia on plasma volume Erythropoietin, HIF, and other stimuli to production High altitude and serum EPO concentration Intermittent hypoxia and EPO concentration Lowlanders going from sea level to high altitude Long-term high altitude residents Optimum hemoglobin concentration Effect of blood boosting on performance at sea level and high altitude23 Erythropoietin and hemoglobin concentration on descent from high altitude23 Iron and hematologic responses to high altitude Platelet function at high altitude The coagulation system at high altitude Following ascent to high altitude, barometric pressure decreases, thereby leading to a decrease in the arterial partial pressure of oxygen and, as a result, a decrease in hemoglobin-oxygen saturation. Acutely, cardiac output increases and helps maintain oxygen delivery in the face of these changes, but over time, the primary compensatory mechanism is a rise in blood hemoglobin concentration and the subsequent increase in oxygen-carrying capacity and arterial oxygen content. This chapter examines the primary factors responsible for changes in plasma volume (PV), which play a large role in the initial increase in hemoglobin concentration (Hb), and changes in red cell mass (RCM), the factor responsible for the continuing rise in oxygen carrying capacity over the following weeks to months at high altitude. The regulation of each of these factors is discussed, as well as how the observed responses are altered by acute and chronic hypoxic exposure, exercise, and other variables, and how these responses may vary between high altitude populations. The chapter concludes with consideration of other aspects of hematologic function at high altitude including platelet function, the clotting cascade, and white blood cell function. As noted above, the initial increase in hemoglobin concentration following ascent occurs as a result of a reduction in plasma volume rather than erythropoiesis itself, as the latter requires several days to weeks to take place. As a result, it is necessary to begin a discussion of hematologic responses to acute hypoxia with a discussion of how plasma volume changes at high altitude and in response to other factors such as changes in posture and exercise. Plasma volume is primarily controlled by a feedback loop involving atrial natriuretic peptide (ANP). This hormone is released in response to increased right atrial stretch (Laragh 1985), which occurs as a result of redistribution of blood volume from the periphery or by an increase in the total blood volume. ANP causes sodium and water loss from the kidneys, which, in turn, reduces PV. The regulation of this system is described in Figure 13.1, although the precise mechanisms by which PV is controlled in acute hypoxia has not yet been elucidated. The endocrine response to high altitude, including ANP, is described in further detail in Chapter 16. Figure 13.1Factors affecting plasma volume (PV) and its regulation by atrial natriuretic peptide (ANP), antidiuretic hormone (ADH), aldosterone (Aldo), and vascular capacity. Na, sodium. Hydration and dehydration obviously affect PV, along with all other body fluid compartments. Depending on whether dehydration occurs by decreased fluid intake or fluid loss via increased diuresis and sweating with its associated salt and electrolyte losses, it may affect the balance of water loss from various body compartments. The vascular capacity is determined by the tone of the vessels, especially the venous capacitance vessels and vessels in the skin. Vascular tone, in turn, depends on a number of factors, such as temperature and catecholamine levels, as well as hydration status. Peripheral vasoconstriction shifts blood from the periphery to the central circulation, raising right atrial pressure, stretching the atria, and stimulating ANP release, while vasodilatation has the opposite effect. A change in vascular capacity also has a more direct effect on PV by shifting the balance of forces in the Starling equation. Vasodilatation tends to reduce the intravascular pressure, favoring inward movement of fluid to the circulation, while vasoconstriction has the opposite effect. Secretion of antidiuretic hormone (ADH) in response to increased plasma osmolality raises PV by decreasing free water loss in the kidney. Increases in vascular volume caused by hydration cause a fall in plasma osmolality that inhibits ADH secretion. This leads to a water diuresis, which leads to an increase in plasma osmolality and a subsequent rise in ADH levels. High sodium intake can cause water retention and increased PV, particularly when intake exceeds the individual’s ability to excrete sodium via the urine and sweat. Similar changes can be seen with sodium retention caused by stimulation of the renin-angiotensin-aldosterone system as a result of exercise or assumption of an upright posture. Exercise and postural changes affect PV via other mechanisms that are discussed further below. These effects are mitigated to some extent by the rise in ANP that follows the increase in PV and the increase in right atrial stretch. Other factors that shift blood volume to the central circulation causing increased atrial stretch and ANP secretion include splanchnic vasoconstriction, assumption of the supine posture, lower body immersion, G-suits, and microgravity experienced by astronauts. Conversely, the upright position tends to shift volume to the lower extremities, reducing right atrial pressure and inhibiting ANP release. The effect of posture must be taken into account when considering the effect of other variables such as hypoxia or exercise on PV. Seventy percent of the blood volume is below the heart in the upright position and, of this volume, 75% is in the distensible veins. Upon standing, 500 mL of additional blood enters the legs. In individuals with intact autonomic function, this leads to a reflex tachycardia and vasoconstriction that are essential to maintain cerebral perfusion and prevent fainting. Vasoconstriction maintains the blood pressure and reduces flow, especially to the skin, muscles, kidneys, and abdominal viscera. The capillaries are exposed to the hydrostatic pressure of the column of venous blood. Upon standing, the height of the venous column is increased, leading to higher hydrostatic pressure, increased filtration of fluid out of the vascular compartment, and, as a result, hemoconcentration. Numerous investigators from Thompson et al. (1928) onward have confirmed these theoretical expectations. Thompson et al., for example, found a reduction of plasma volume of 15% on assuming the upright position, but the magnitude of this effect is variable and is influenced by many factors, including the temperature of the environment and the subject, the state of hydration, etc. Exercise has an important effect on plasma volume and, therefore, hemoglobin concentration, with the effect varying according to the intensity, duration, and type of exercise, the temperatures of the environment, and the posture assumed during exercise. This is because temperature and posture affect the skin blood flow and hence the distribution of cardiac output to the working muscles and other vascular beds. This, in turn, affects the capillary and venous pressures in these areas and hence the balance of forces in the Starling equation. Many studies on the effect of exercise have ignored the effect of posture and have taken control samples in a different posture from exercise samples. Another important factor that may affect PV is the high insensible fluid losses, particularly through the respiratory tract, as a result of the increased minute ventilation. These factors play a significant role at high altitude where humidity falls and minute ventilation is higher for any given level of work than at sea level. Harrison (1985) reviewed the literature and, with a number of reservations, came to the conclusion that, for bicycle ergometer exercise, there is a reduction in PV soon after starting exercise. This reduction is proportional to the intensity of exercise or, more precisely, to the rise in atrial pressure. Thereafter, there is little change with continued exercise at normal room temperature, but in high temperatures, there is a further reduction in PV with time due to sweating. However, these laboratory studies tend to look at fairly high intensity exercise (greater than 50% V.O2max) for periods of up to an hour or two. Exercise in the mountains, on the other hand, is typically done over many hours and may go on day after day. Exercise of eight hours or more at normal climbing rates (i.e., up to about 50% V.O2max but often averaging much less) is associated with an increase in PV. Pugh (1969), for example, found an increase in blood volume of 7% after a 28-mile hill walk, while Williams et al. (1979) found PV increased progressively for five days of strenuous daily hill walking, eventually reaching a value 22% above the baseline value. Both these studies were carried out under cold conditions and subjects avoided both overheating and cold stress. The changes in PV, interstitial, and intracellular volumes documented in the study by Williams et al. are shown in Figure 13.2. The reason for the expanded PV after sea-level mountaineering is predominantly due to the effect of exercise. The mechanism for these exercise-induced changes in PV is due, in part, to activation of the renin-angiotensin-aldosterone system (Milledge and Catley 1982), although multiple other factors play a role as well (Montero and Lundby 2018). Figure 13.2The effect of five consecutive days of strenuous hill walking on body fluid compartments. The changes are calculated from changes in packed cell volume and sodium and water balances. (Source: Williams et al. 1979.) It should also be noted that prolonged, continuous climbing increases the risk of dehydration and reduction in PV. In many cases, however, adequate fluid supplies are available and the risk of dehydration and heat illness remains low as long as the individual is conscientious about maintaining adequate fluid intake. Within one to two hours of exposure, mild-moderate degrees of acute hypoxia trigger diuretic and natriuretic responses in the kidney that can last for one to two days, while more severe levels of acute hypoxia (FIO2 <0.10) may actually be associated with antidiuretic responses and sodium retention (Swenson 2001). The diuretic and natriuretic responses seen with mild-to-moderate exposures subsequently reduce PV over the first several days at altitude, although the effect of hypoxia in field studies may be overshadowed in some cases by the effects of cold, dehydration, and exercise. Singh et al. (1990), for example, found a reduction in PV from 40.4 mL kg−1 at sea level to 37.7 mL kg−1 on day 2 at 3500 m, and 37.0 mL kg−1 on day 12. Wolfel et al. (1991) reported similar changes in PV on ascent to 4300 m, with PV falling from 48.8 mL kg−1 to 42.5 mL kg−1 on arrival at altitude and 40.2 mL kg−1 by day 21, while Robach et al. (2002) demonstrated a 13.6% reduction in plasma volume after seven days at 4350 m. It should be noted, however, that the reported reductions in PV may not apply in all circumstances. Subjects who develop acute mountain sickness, for example, may actually experience antidiuresis and expanded PV. Vigorous exercise on the way up to high altitude or following arrival can also result in expansion of the PV, as it does at sea level. Withey et al. (1983), for example, found that subjects who hiked up to the Kulm Hotel on the Gornergrat (3100 m) and continued to exercise with hill walking thereafter had an increased PV and decreased hematocrit on the second day after ascent. In this particular situation, the effect of exercise overrode the effect of hypoxia. Some caution must be exercised in the interpretation of these and other studies in light of the technical limitations surrounding equation-based modelling on hematocrit and hemoglobin as demonstrated in the Dill and Costill method and the Evans’ Blue approach. For example, Poulson et al. (1998) measured the change in PV of 10 subjects airlifted to the Observatoire Vallot (4350 m) using both the Evans’ blue and the carbon monoxide rebreathing methods. Twenty-four hours after arrival at altitude, they found the expected reduction in PV with the carbon monoxide method (350 mL reduction) but not with the Evans’ blue method (30 mL reduction). A possible explanation is that, since the Evans’ blue method labels albumin, and hypoxia leads to increased capillary permeability to albumin, the Evans’ blue method may include this extravascular pool of albumin and thus give a falsely high result. Given that the carbon monoxide rebreathing method can be implemented in most populations on a frequent basis (∼12–24 hour washout), and with an exceptionally high accuracy (Pottgiesser et al. 2007; Thomsen et al. 1991) and precision (typically 1.5–2%), future research in this area is likely to improve substantially. One caveat is that while this procedure is simple for the participant, technical details must be followed precisely to obtain accurate results (Siebenmann et al. 2017a). Following the early phases of high altitude exposure, acclimatized lowlanders experience a reduction in PV over the next few weeks. Pugh (1964b) found a 21% reduction in PV after 18 weeks at altitudes above 4000 m in four members of the 1960–61 Silver Hut Expedition. During the following seven to 14 weeks, PV returned toward control levels, with values being on average 10% less than control when corrected for changes in body weight. Changes in plasma and blood volume over time at altitudes ranging from 2900 m to 5000 m have been reviewed (Siebenmann et al. 2017b) (Figure 13.3) and a range of potential underlying mechanisms identified (Figure 13.4). Figure 13.3Changes in plasma (A) and total blood volume (B) throughout exposure to different altitudes. A: data from 21 peer-reviewed articles; B: data from 16 peer-reviewed articles. Small-, middle-, and large-sized data points illustrate results collected in 1–3, 4–7, and 8–10 subjects, respectively. Points connected by straight lines represent the weighted average for the two altitude categories calculated over 1–5, 6–10, 11–15, 16–20, and 21–30 days, respectively. In B, no weighted average is presented for 21–30 days at 4000–5000 m due to the limited available data. (Source: Siebenmann et al. 2017b.) Figure 13.4Summary of changes in intravascular volume at high altitude and potential underlying mechanisms. Diuresis and erythropoietin are highlighted since they are considered the cardinal mechanisms underlying plasma volume contraction and total red blood cell volume expansion, respectively. PV, plasma volume; RCV, total red blood cell volume; R-A-A, renin-angiotensin-aldosterone axis; ADH, antidiuretic hormone; ANP, atrial natriuretic peptide. (Source: Siebenmann et al. 2017b.) With regard to long-term residents, Sanchez et al. (1970) found that residents of Cerro de Pasco, Peru (4370 m), had mean PV two-thirds that of a group of students at Lima (sea level). When allowance was made for the weight difference of the groups, they still had a PV 27% less in a blood volume that was 14% greater. This effect has been measured by others (Gamboa et al. 2006) and recently extended to Sherpa who surprisingly have a larger plasma volume compared to acclimatized lowlanders and Andeans. Indeed, this expanded plasma volume maintains normal hematocrit values and masks a significantly larger red cell mass (Stembridge et al. 2019), a physiological consequence of chronic hypoxia thought not to occur in Sherpa based on measurements of hemoglobin alone (see next section). Plasma volume rapidly returns to baseline levels upon return to sea level. In Operation Everest III, PV was, on average, higher than before altitude exposure after only one to three days at sea level (Robach et al. 2000), while, in the study noted earlier by Robach et al. (2002), PV returned to control values within 24–30 hours of returning from 4350 m to sea level. In the latter study, the observed change was attributed to decreased diuresis stemming from changes in fluid-regulating hormones, such as ADH, aldosterone, ANP, and renin. The lower plasma volume seen in Andeans increases after relocation to sea level (Gamboa et al. 2006). Red cell mass is determined by the rate of formation of red cells (erythropoiesis) and their rate of loss. Red blood cells are generally lost by their death (their natural length of survival is about 120 days) but can also be lost as a result of hemorrhage, pathological states such as hemolytic anemia, and targeted destruction of typically young red blood cells, referred to as neocytolysis (Mairbaurl 2018). Erythropoiesis is impaired by bone marrow disorders, chronic kidney disease, or deficiencies in important cofactors necessary for hemoglobin synthesis, including iron or vitamin B12. In the absence of these problems, erythropoiesis is controlled by the concentration of the hormone erythropoietin (EPO). EPO is produced primarily by peritubular cells in the kidney, although 10–15% of total production occurs in the liver (Erslev 1987). The gene coding for the hormone has been cloned and expressed in cultured cells, allowing for sufficient material to be produced for clinical use, such as management of anemia in patients with chronic kidney disease. The two classical stimuli for EPO secretion are hypoxia and blood loss, both of which result in tissue hypoxia. In terms of evolutionary pressures, blood loss is probably more important than hypoxia, as blood loss is a far more common threat to survival than chronic hypoxia and erythropoiesis is far too slow a process to adequately defend against acute hypoxia. However, another important regulator of EPO production and red cell volume is exercise training. While the exact mechanism(s) are not completely understood (Montero and Lundby 2018), it is an important factor to consider in any scientific study that involves trekking/mountaineering, especially in previous untrained people. Oxygen-sensing cells in the inner cortex and outer medulla of the kidney respond to tissue hypoxia and regulate EPO production and secretion (Semenza 1994; Semenza 2009). This was nicely demonstrated by Fisher and Langston (1967), who perfused an isolated dog kidney with hypoxic blood and noted a rise in EPO concentrations. There are several important differences between this oxygen-sensing pathway and another important oxygen-sensing system, the carotid body, which mediates the hypoxic ventilatory response (HVR). While HVR occurs within seconds following a step change in arterial PO2, there is no detectable rise in EPO concentration for over an hour after exposures to 3000–4000 m (Eckardt et al. 1989) and full EPO responses occur over several days (Semenza 2009). Moreover, the oxygen-sensing cells in the kidney respond to oxygen content, unlike the carotid body, which is sensitive to reduction in PO2. As a result, anemia leads to a rise in EPO concentrations, whereas it has no effect on the carotid body and the hypoxic ventilatory response. However, it appears likely that both responses in the kidney and the carotid body are comprised of an integrated upregulation of the hypoxia-inducible factor (HIF) system (Prabhakar and Semenza 2016; Schodel and Ratcliffe 2019). Erythropoietin is one of a number of gene products whose transcription is stimulated by hypoxia, including enzymes involved in glycolysis (aldolase A, enolase-1 glucose transporter-1, lactate dehydrogenase, and phosphofructokinase), mediators of vascular tone (inducible nitric oxide synthase and heme oxygenase), and factors involved in angiogenesis (vascular endothelial growth factor). The links between hypoxia and gene induction for all these proteins are the hypoxia-inducible factors, HIF-1α and HIF-2α. First identified as a nuclear factor that bound to the hypoxia response element of the EPO gene (Semenza 1998), HIF is normally produced and rapidly degraded in normoxia, but accumulates in hypoxia and exerts many important downstream effects on gene regulation, which are described in Chapter 6 and reviewed in detail elsewhere (Schodel and Ratcliffe 2019). Although hypoxia is a major stimulus for EPO production, other stimuli have been investigated. Conflicting evidence has been reported regarding the effect of exercise with some studies reporting a rise in serum erythropoietin with exercise (Montero et al. 2017; Schwandt et al. 1991) and others finding no effect (Bodary et al. 1999; Schmidt et al. 1991). Roberts et al. (2000) investigated whether plasma volume affects EPO production. They measured serum concentrations before and after supramaximal exercise and after 5% PV reduction by plasmapheresis. Both exercise and PV reduction caused a statistically significant increase in EPO. Other stimulants to EPO production may be the plasma thiol-disulfide redox state and inert gases such as xenon. Using a crossover design, Hildebrandt et al. (2002) demonstrated that the EPO response to two hours of hypoxia was greater following treatment with the antioxidant N-acetyl-cysteine than following treatment with placebo, while Dias et al. (2019) noted a small rise in EPO concentration following very short (minutes) periods of xenon inhalation. Interestingly, seven consecutive days of xenon inhalation caused a small rise in EPO and a substantial plasma volume expansion. Until about 1980, measurements of blood EPO levels were made by bioassays that could not detect the hormone until the concentration was greater than normal sea level values. Therefore, earlier work often relied on more indirect indices of erythropoietic activity, such as intestinal iron absorption or reticulocyte counts that did not necessarily provide an accurate sense of EPO regulation. Intestinal iron absorption, for example, has been shown to be independent of EPO and to be promoted as a direct effect of hypoxia rather than secondary to plasma iron turnover or erythropoietic activity (Raja et al. 1986), while the reticulocyte count rises many days following EPO secretion due to the typical time frame of red blood cell production in the marrow. Using such methods, early studies showed that following ascent, EPO concentrations rose within the first 24–48 hours (Abbrecht and Littell 1972; Siri et al. 1966). Studies using radioimmunoassay methods sensitive to EPO levels well below the normal range (13–37 mIU mL−1) have shown that serum immunoreactive EPO concentration (SiEp) rises within two hours of hypoxic exposure (Eckardt et al. 1989) and reaches a maximum at about 24–48 hours. Thereafter, it declines to values not measurably different from sea level after about three weeks, as shown in Figure 13.5 (Milledge and Cotes 1985). Gunga et al. (1994) have reported similar findings at a more modest altitude of 2315 m. Even after three weeks above 4500 m, short-duration gains in elevation to 5500 m lead to an increase in SiEp, which persists even after return to lower elevation (Figure 13.5). A similar rise in SiEp following restoration of normoxia was shown by Knaupp et al. (1992) who demonstrated a 50% increase in SiEP levels 240 minutes after starting a 120-minute exposure to an FIO2 of 0.1. The apparent rise in SiEp after descent or restoration of normoxia likely reflects the time delay necessary for gene transcription and protein production. One of the noteworthy features of the observed EPO responses is the significant degree of interindividual variability. Richalet et al. (1994) studied subjects over a prolonged stay at 6540 m and documented anywhere from threefold to 134-fold increases in SiEp values. Figure 13.5The effect of going to high altitude on the serum erythropoietin concentration. The top panel shows the altitude/time profile for the eight subjects. The dotted line indicates ascent above base camp between blood samples. Note, the samples at 30 days were taken at 5500 m after four sample times at base camp (4500 m). Mean packed cell volume (PCV) is shown in the center panel and mean erythropoietin concentration in the lower panel. C, control, sea level; K, Kashgar (1200 m); L, Karakol lakes (3500 m). (Source: Milledge and Cotes 1985.) With persistent hypoxic exposure, EPO levels decrease in response to the rise in hemoglobin concentration. Wedzicha et al. (1985), for example, showed that in patients with polycythemia secondary to hypoxic lung disease, SiEp was within the normal range in more than 50% of patients. A study in mice at simulated high altitude found that if the increase in hemoglobin concentration ([Hb]) is prevented by either phlebotomy or treatment with the hemolytic drug, phenylhydrazine, SiEp remained elevated for the 10 days of the study, whereas in control mice, there was the usual fall on the second and subsequent days to levels about twice that of baseline values (Bozzini et al. 2005). This study suggests that the normal increase in [Hb] on ascent to high altitude is sufficient to relieve, to a degree at least, the tissue hypoxia causing the rise in SiEp and provides confirmation in very controlled conditions of an incidental finding from the Sajama Expedition (Richalet et al. 1994) in which two female team members who showed no increase in [Hb] after 10 days at 6542 m had large increases in SiEp compared with the other subjects. While EPO was measured after continuous hypoxic exposures in the studies noted above, it is also important to consider what happens with intermittent exposures, particularly given the increasing interest in intermittent hypoxia and its role improving athletic performance as well as in in the pathophysiology of diseases, such as sleep-disordered breathing. Whereas some studies have shown increases in SiEp (Gore et al. 2006; Koistinen et al. 2000), other studies have shown no change in EPO levels following the intermittent hypoxic exposure (Julian et al. 2004; Lundby et al. 2005). The variability in study results may relate to the fact that the different studies typically involve widely divergent protocols for what is considered “intermittent” hypoxia. Gore et al. (2006), for example, exposed subjects to a simulated altitude of 4000 m to 5000 m for three hours a day, five days a week for a total of four weeks, while Julian et al. (2004) exposed subjects to 70-minute episodes of alternating hypoxia (five minutes) and normoxia (five minutes) over a four-week period. Because the life span of the red cell is unchanged at high altitude (Berlin et al. 1954), increased erythropoiesis leads to an increase in red cell mass (RCM). As shown in Figure 13.6, the rise in red cell mass is slow, but continues for a long time, increasing by a mean of 50% in absolute terms or 67.5% when corrected for loss of body weight after about six months above 4000 m. By this time, the blood volume had increased over control by 7.3%, or 22.8% corrected for body weight (Pugh 1964a). Similar changes are seen in high altitude residents. Sanchez et al. (1970), for example, found high altitude residents in the Andes to have an RCM 83% greater than sea-level residents when corrected for weight difference. Figure 13.6Changes in hemoglobin concentration (Hb%), red cell volume, blood volume, and plasma volume in four subjects during the Silver Hut Expedition: (a) after 19 weeks at between 4000 m and 5800 m; (b) after a further three to six weeks at 5800 m; (c) after a further nine to 14 weeks at or above 5800 m. (Source: Pugh 1964a.) The combined effect of changes in PV and RCM results in an increase in [Hb]. As discussed earlier, the initial rise in [Hb] during the first few days and weeks at high altitude is largely a result of reduction in PV. The [Hb] rise is roughly exponential with time, leveling out at about six weeks at a given high altitude. RCM continues to rise after that time, but PV rises as well and, as a result, [Hb] remains approximately constant (Figure 13.6). Some of the clearest evidence of the changes in [Hb] over time at high altitude comes from Pugh (1964c), who reviewed results from five expeditions (51 observations in 40 subjects) and concluded that the [Hb] averaged 20.5 g dL−1 after about six weeks at high altitude; there was no correlation between [Hb] and performance on the mountain. Reviewing [Hb] values from the 1981 American Medical Research Expedition to Everest and two previous Everest expeditions, Winslow et al. (1984) found the range of mean values was 17.8–20.6 g dL−1 at altitudes of 5350–6300 m, with no correlation between high altitude and [Hb] within this altitude range. The increase in [Hb] allows more oxygen to be carried per liter of blood at any given oxygen saturation. Beyond a certain point, however, the benefits of increasing oxygen-carrying capacity may be offset by the increases in blood viscosity (discussed further below). Concern has been expressed in the past that the increase in viscosity may predispose to thrombosis at altitude but there is no systematic evidence to support either this claim or the fact that the risk of venous thromboembolism is increased at altitude (Chapter 23). In general, the average [Hb] among long-term residents increases with increasing altitude (Figure 13.7), with a noteworthy feature of this relationship being the variability in [Hb] across the different high altitude populations. Early reports demonstrated that Andean subjects, for example, had values in the region of 22 g dL−1 at altitudes of 4300–4500 m (Dill et al. 1973; Talbott and Dill 1936). These studies may have included subjects who would now be considered to have chronic mountain sickness, and if one focuses instead on healthy individuals, the mean values are slightly lower than the earlier data. In a comprehensive study of hematologic parameters of high altitude residents in the Andes, for example, Leon-Velarde et al. (2000) reported mean [Hb] of 18.5 g dL−1 and 20.4 g dL−1 in adult men (40–60 years old) living at 4355 m and 5500 m, respectively. Women in the same age range had mean values of 17.5 g dL−1 and 18.1 g dL−1 at the same altitudes. The authors also showed that the average [Hb] increased with increasing age at each elevation. Women had similar values as men before puberty while older women had higher values than younger women, likely due to the absence of menstrual periods following menopause. Figure 13.7Metaregression analysis of changes in the hemoglobin concentration with altitude of residence in adult females (A) and males (B). Data are grouped by ethnicity or region/country. Each point indicates the mean value of a dataset reported in the literature. The size of each dot reflects its precision and thus its influence on the regression. Lines are shown where significant (full lines) or where a trend (P <0.1; dashed line) of differences to the reference group (the American females; gray line) existed in intercept or slope. (From Gassmann et al. 2019.) Other high altitude populations have been shown to have lower [Hb] than the Andean residents. Adams and Strang (1975) reported a mean of 17 g dL−1 in Sherpas living at 4000 m, while Morpurgo et al. (1976) reported a mean value of 16.2 g dL−1 and argued that it represents greater adaptation. Beall and Goldstein (1987) studied Tibetans and demonstrated a hemoglobin concentration of 18.2 g dL−1 in male and 16.7 g dL−1 in female subjects resident at an altitude of 4850–5450 m, a value substantially lower than most results from the Andes at comparable altitude. In a later study, Beall et al. (1998) studied highland populations at high altitude in Tibet and Bolivia and found Tibetans had significantly lower hemoglobin concentrations than the Bolivian highlanders (15.6 g dL−1 compared to 19.2 g dL−1). The results of this study agree with those of Winslow et al. (1989) who compared Himalayan natives (Sherpas) to high altitude Andean natives at similar altitudes in Khundi, Nepal, and Ollague (Chile) at 3700 m and reported that packed cell volume (PCV) values in Nepal were significantly lower than in Chile (48.4 g dL−1 compared with 52.2 g dL−1). They also found serum EPO concentrations to be higher in the Andean population, indicating that they were functionally anemic even with the higher PCV. High altitude Ethiopian residents may have similar [Hb] to the Tibetans. Beall et al. (2002) examined residents at 3530 m and found mean [Hb] of 15.9 g dL−1 and 15 g dL−1 for men and women, respectively, while Hoit et al. (2011) reported mean [Hb] of 16.3 g dL−1 and 15.3 g dL−1 in Amhara men and women, respectively, living at 3700 m in elevation. Finally, Caucasian individuals living in high altitude towns in Colorado and acclimatized climbers tend to have lower hemoglobin concentration than Andeans. The reasons for the observed differences between these populations are not entirely clear and may be related to a variety of factors. One important factor may be the time that a given population has spent at high altitude. It is estimated that Tibetans have been resident at high altitude for at least ∼30,000–40,000 years, compared with ∼7000–11,000 years for Andean highlanders. Support for this concept comes from a large study (N = 5887) by Wu et al. (2005) from northwest China and Tibet in which they compared [Hb] in Tibetans with [Hb] in lowland Han Chinese who had relocated to Tibet and remained there for many years. The rise in [Hb] with high altitude in Han Chinese follows the same line as that given by a regression equation derived from the literature for North and South American populations at various altitudes. This rise is 1 g dL−1 for every 1100 m increase in altitude (y = 1.1x + 14.0 when altitude is given in kilometers) but the [Hb] was considerably higher in this group when compared to the Tibetans, who showed only a very small rise with altitude increase from 3813 m to 5200 m. The time spent at high altitude is likely providing an opportunity for selection pressures and genetic adaptation to the environment. In their study of Tibetan and Bolivian highlanders, Beall et al. (1998), for example, found that genetic factors accounted for a very high proportion of the phenotypic variance in hemoglobin concentration in both samples. Newer studies have provided evidence for this concept. Beall (2011) showed that variation in genes encoding transcription factors for HIF-2α explained observed differences in [Hb] between Tibetan highlanders and ethnically similar Han Chinese who had relocated to Tibet. Two other studies published at the same time yielded similar findings and provided further strong support for the role of genetic adaptation (Simonson et al. 2010; Yi et al. 2010). Despite these observations, one important feature is that most data presented in this section are based on [Hb] in g dL−1 and thus substantially affected by plasma volume and not placed in the overall context of total blood volume. Indeed, based on recent data, it seems that natural selection and genetic adaptation in Tibetans favors larger total blood volume and not [Hb] per se (Stembridge et al. 2019). Other factors that could explain some of the variation might include the fact that some of the groups, such as the Colorado residents, move up and down in altitude more frequently than the Andeans who tend to remain on the Altiplano, the fact that Colorado residents reside at an altitude that is typically 1000 m lower than Andeans, or dietary factors such as the level of meat and, therefore, iron in the diet. Excessive rise in [Hb] (i.e., above 22 g dL−1) is generally considered to be pathological and diagnostic of chronic mountain sickness (Chapter 24). Both people native to high altitude, such as the Andean populations, and lowlanders resident at high altitude for many years, such as the Han Chinese who relocated to the Tibetan plateau (Huang et al. 1984) are at risk of developing this condition. The increase in [Hb] increases the oxygen-carrying capacity and oxygen content of blood and, as a result, compensates for a reduction in arterial PO2 and oxygen saturation. At altitudes up to about 5300 m, the arterial oxygen content in acclimatized individuals is approximately equal to that at sea level (Figure 13.8). However, increasing [Hb] results in increasing viscosity (Guyton et al. 1973). This increase in viscosity is curvilinear so that, with [Hb] above about 18 g dL−1, viscosity increases rapidly. Eventually, this increased viscosity increases resistance in both systemic and pulmonary circulation, leading to reductions in pulmonary and systemic blood flow and a drop in cardiac output. With the reduction in cardiac output, oxygen delivery, the product of cardiac output and arterial oxygen content, decreases, thereby impeding oxygen supply to the tissues.
Introduction
Effect of High Altitude on Plasma Volume
Regulation of plasma volume
Hydration Status
Vascular Capacity
Antidiuretic Hormone
Sodium Status
Other Factors
The effect of posture on plasma volume
Exercise and plasma volume
Effect of acute hypoxia on plasma volume
Effect of chronic hypoxia on plasma volume
Plasma volume on return to sea level
Erythropoiesis
EPO and its regulation
Erythropoietin, HIF, and other stimuli to production
High altitude and serum EPO concentration
Intermittent hypoxia and EPO concentration
High altitude and red cell mass
Hemoglobin Concentration
Lowlanders going from sea level to high altitude
Long-term high altitude residents
Polycythemia of high altitude
Optimum hemoglobin concentration
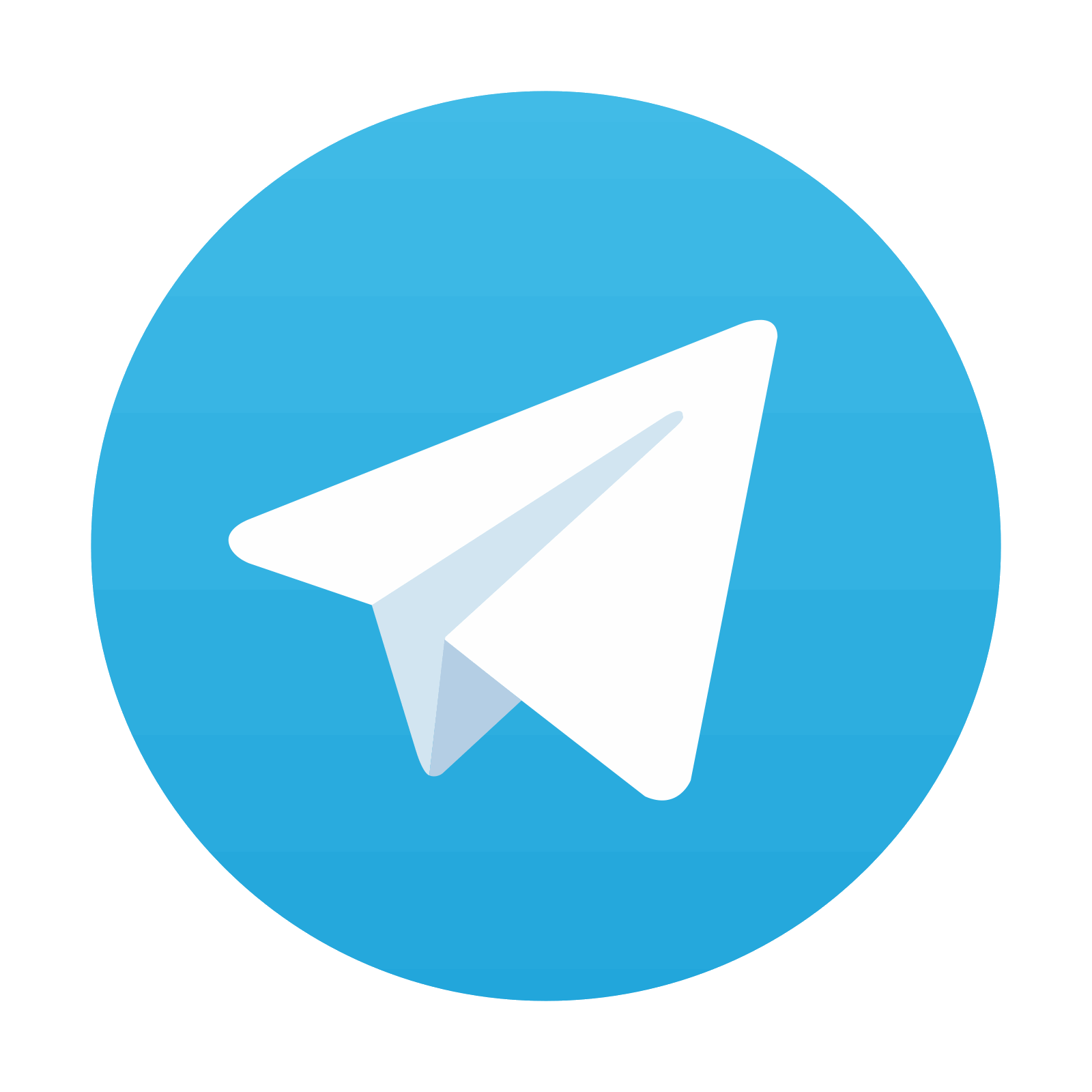
Stay updated, free articles. Join our Telegram channel
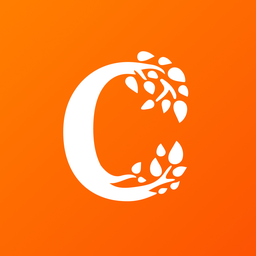
Full access? Get Clinical Tree
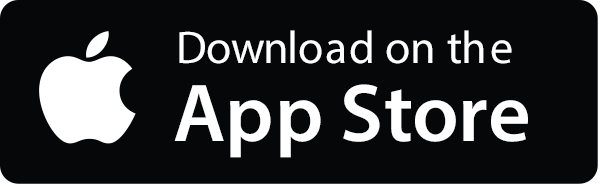
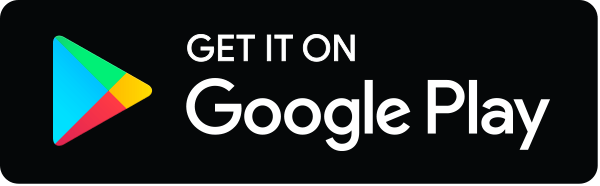