Fig. 10.1
Several age-related changes are shown in this image. (a) The impact of aging on neurotransmitter receptors is illustrated. (b) AP traces of both young and aged neurons are illustrated. (c) Age-related changes as seen by the right side of this section show translocation of potassium receptors. (d) Age-associated myelin depletion is seen compared to the healthy neuron shown on the left side of this image. (e) Receptor types and properties also are shown to change with age (Reprinted from Rizzo et al. [19])
Years later, neuroimaging studies demonstrated that after middle age, there is increased neural recruitment compared to adolescence and young adulthood [21]. This increased neural recruitment has been interpreted as a compensatory response to decreased neural network integrity in older adults [22–26]. This hypothesis has been supported by prospective trials that found greater engagement in prefrontal and hippocampal regions in older adults performing memory tasks [27, 28]. This concept (i.e., “less wiring more firing”) has also been extended to motor pathways [29].
The regulation of cerebral vasculature is similarly impacted by the passage of time; after middle age, patients are susceptible to a decline in cerebral blood flow and cerebral blood velocity [30]. There is limited data to determine the extent to which there are age-dependent changes in cerebral metabolism or autoregulation. Nonetheless, a prospective study from 2003 determined that dynamic cerebral autoregulation remains preserved in healthy people above age 60 after undergoing a 30-min tilt test [31, 32].
Additional changes in cerebral physiology are seen in the regulation of CSF production and turnover, and in the function of the choroid plexus and blood brain barrier (BBB) . The choroid plexus plays a pivotal role in neuronal development early in life by supplying trophic factors needed for growth and differentiation . In adulthood, it helps maintain homeostasis within a mature nervous system by helping regulate transport across the BBB, repairing injury with the presence of neuro-progenitor cells and clearing toxins. CSF turnover diminishes in older adults and thus hinders clearance of metabolic waste and transport of bioactive nutrients. Indeed increased CSF volume has been associated with neurocognitive impairment [20, 33]. With senescence, there is also increased leakage in the BBB as confirmed by brain imaging [34]. Rodent models suggest that this may be a function of oxidative stress, increased permeability to TNF-alpha, decreased presence of GLUT-1 glucose transporter expression, altered iron accumulation, and hormonal imbalances [35–39].
Biochemical Changes (Neurotransmitter Associated)
Whereas early studies stated that neurotransmission was largely preserved with aging [40], technological advances in neuro-imaging and neuroreceptor ligand binding unearthed alterations in neurochemistry and signaling through several key neurotransmitter receptors. Key age-dependent changes in specific neurotransmitters and their cognate receptors are detailed below. It is important to note that our knowledge remains incomplete in this area; future studies will be necessary to more fully understand age-related changes in neurotransmission.
Serotonin
Serotonin is well known to play a pivotal role in memory formation, emotion and mood regulation, sleep homeostasis, and pain modulation. Additionally, serotonin plays a key role in platelet biology, gastric motility regulation by the enteric nervous system, and numerous other physiologic processes outside of the nervous system [41]. There appears to be a preservation of serotonin content and innervation with time, but neurochemical and PET imaging reveals an age-dependent decrease in cortical serotonin receptor numbers. Therefore, while neurochemical deficits are not present, there is the potential for impaired neurotransmission [42, 43]. Though direct clinical implications have yet to be identified, it is reasonable to suspect this may play a role in mood modulation and sleep dysfunction seen in the elderly, as similar symptoms are often seen in depressed adults. Given the success of selective serotonin reuptake inhibitors (SSRIs) in treating depression, it would be worthwhile to study the implications of SSRIs on aging individuals both with and without depression. Would supplemental serotonin by any means alter progression of memory impairments? Would this help prevent depression in the elderly? There is evidence that use of SSRIs poststroke improves physical recovery, presumably by enhancing neuronal plasticity and neurogenesis [44]. Data also suggests that SSRIs mediate hippocampal neurogenesis, potentially enabling plasticity to help build a more productive pattern of thought and behavior [45]. Additionally, further studies will be needed to help appreciate the pharmacological adjustments required when prescribing SSRIs for older adults. While one could argue that aging individuals may need higher doses due to their inherent impairments in serotonergic neurotransmission, their aging renal and hepatic systems may suggest lower dose requirements for a similar clinical impact.
Dopamine
Dopamine receptors are found in the central nervous system in addition to the cardiovascular, pulmonary, gastrointestinal, and renal systems. In the central nervous system, dopamine is primarily localized to the striatum and implicated in motor and cognitive functions such as reward processing, memory encoding/retrieval, and verbal fluency. Dopamine is also instrumental in communication between the ventral tegmental area and nucleus accumbens, a central reward processing unit responsible for positive reinforcement and reward behavior [46]. Key neural receptors are D1 and D2; whereas both are postsynaptic receptors, there are also presynaptic D2 receptors that are instrumental for autoregulation of dopamine release. There is a significant age-related decline in dopamine concentration and dopamine receptor numbers [47–49]. This downregulation is thought to be secondary to age-related loss of dopaminergic synapses and neurons; however, the mechanism behind these changes is unresolved and likely multifactorial [50]. Regardless, the changes likely contribute to diminished cognition and motor performance in older adults [51–53]. Presumably, these changes can also lead to increased susceptibility to anhedonia and altered affective responses to normally rewarding stimuli in older adults.
Acetylcholine
Acetylcholine is ubiquitous in the peripheral and central nervous system. Nicotinic and muscarinic acetylcholine receptors are found in both the brain and periphery, and play a key role in higher cognitive functions, the autonomic nervous system and the neuromuscular junction. M1 receptors are typically found in the cortex, hippocampus, nucleus accumbens, globus pallidus, and caudate nucleus. M2 receptors are largely present in the thalamus, brain stem, pons, and cerebellum. An evaluation of 58 postmortem human brains revealed that muscarinic receptor expression decreased in the frontal regions (largely M1) and increased in the thalamus (mainly M2) with healthy aging. To evaluate nicotinic receptors, the group used two different ligands: nicotine and acetylcholine. Nicotine binding-associated data showed regional changes similar to those seen with muscarinic receptors; however, no significant age-related changes were seen with acetylcholine as a ligand. The investigators attributed this disparity to nicotine having increased binding sites and changes in receptor subtypes with aging [54]. In addition to changing receptor densities, there is likely a decrease in cerebral choline uptake with age even in the presence of increased plasma choline [55]. These changes depict diminished cholinergic function with aging, highlighting the need to avoid anticholinergic medication in already cholinergic-deficient older patients. Anticholinergic medications worsen cognitive function and can precipitate postoperative delirium in the elderly [56, 57].
NMDA Receptors
N-methyl D-aspartate (NMDA) receptors regulate learning, memory, and synaptic plasticity and are largely localized to the hippocampus and cortex. The NMDA receptor has multiple ligands including glycine, glutamate, zinc, and magnesium. Although there are multiple NMDA receptor subunits for the NUMDA receptor, the GluN1 and GluN2 subunits have been most extensively studied and show strong homology between rodents and humans. Early studies indicated an age-related decrease in receptor density for these subunits and NMDA binding with associated impairments in hippocampal-dependent memory function [58]. These effects can be attenuated by environmental changes such as dietary supplementation (omega-3 fatty acids, ginseng, etc.) and caloric restriction [59]. Despite these neuroprotective factors, there appears evidence that even the receptors that remain function less effectively in memory consolidation than those found in younger individuals [60]. These findings suggest that in addition to age-related decreases in NMDA receptor expression, there are also age-related decreases in downstream intracellular signaling and functional neuronal responses to the remaining NMDA receptors.
GABA
Gamma-aminobutyric acid (GABA) is a key inhibitory neurotransmitter in the mature nervous system, but interestingly is predominantly an excitatory neurotransmitter in the developing nervous system [61]. There are three well-established receptors: GABAA, GABAB, GABAC . However, we will focus on the GABAA receptor, since it is a molecular target of many anesthetics such as inhaled anesthetics, barbituates, etomidate, propofol, and benzodiazepines. The GABAA receptor is composed of five subunits and each distinct five-subunit formation has unique pharmacological and electrophysiological properties. While several configurations are known and have been identified, we will speak of GABAA receptors globally in this chapter. GABAA receptors are distributed throughout the cortex, hippocampus, cerebellum, and inferior colliculus, and play a role in memory formation, sedation, and anxiolysis. Much of our knowledge about the aging nervous system and changes in the GABAA receptor comes from rodent models; it is prudent to recognize that the rodent nervous system may not translate perfectly to our own and, therefore, these studies must be interpreted with caution. Though total GABAA receptor binding does not change with healthy aging, there appears an increase in binding density in the hippocampus [62]. Additionally, there is evidence that benzodiazepines produce a greater GABA-mediated current in cells found in the matured nervous system, signifying that increased sensitivity to benzodiazepines occurs in the elderly due to biochemical changes and not just decreased drug elimination or other pharmacokinetic changes [63, 64]. This information could explain why older patients have an increased sensitivity to other anesthetic ligands of the GABAA receptor.
Histamine
Histaminergic neurons are involved in the sleep wake cycle, temperature regulation, endocrine pathways , cognitive processing, appetite, attention, and memory. Peripherally, they are also involved in chemotaxis, uriticaria, gastric acid secretion, bronchoconstriction, and vasodilation. Within the CNS, histaminergic pathways originate in the tuberomammillary nucleus and project to the hippocampus, cerebral cortex, hypothalamas, amygdala, and nucleus accumbens. While four histamine receptors have been isolated, only three receptors (H1, H2, and H3) appear in the CNS. H1 and H2 pathways affect physiologic functions, memory formation, and emotion regulation. H3 receptors regulate histamine pathways by acting presynaptically and also moderate release of other neurotransmitters such as norepinephrine, acetylcholine, and dopamine [65].
Aging is associated with a decline in H1 binding in the frontal, temporal, and parietal regions by 13% per decade (as measured by positron emission tomography) without any appreciable change in receptor density seen in vitro binding [66]. Though this study did not study the direct clinical impact of their findings, later research revealed that diminished histamine plays a role in the increased cognitive deficits seen in senescence. Additionally, H3 antagonists reverse cognitive deficits in a mouse model of accelerated aging (i.e., the senescence-accelerated mouse), likely by blocking presynaptic H3 receptor-mediated inhibition of histamine release [67]. While the H3 receptor regulates the release of several different neurotransmitters, the simplest interpretation of this study is that increasing histamine release in the aging brain ameliorates age-related cognitive deficits.
Orexin
Orexinergic neurons have their cell bodies in the lateral hypothalamus and play a role in wakefulness, energy balance, and appetite. Orexin is most commonly linked to narcolepsy, where there is a profound loss of orexin production in the brain. Animal models demonstrate a significant loss of orexin production with age with concurrent increases in lethargy, diet-induced obesity, insulin signaling dysregulation, and altered brown adipose tissue thermogenesis [68]. Yet, aged rodents that were given supplemental orexin had a smaller increase in arousal, appetite, and a smaller alteration in their circadian rhythms than younger rodents who received identical supplementation [69]. Taken together, these findings suggest that older animals not only have lower levels of orexin, but are also less sensitive to exogenous orexin. Whether similar changes in orexin biology and physiology occur with age in humans remains to be seen; the recent FDA approval of orexinergic antagonists will likely help us understand this question in humans [70].
Plasticity
Aging is not simply a function of cellular death as previously believed; it is a culmination of structural, biochemical, and physiological changes over time. These changes cannot be simplified as a process of inevitable decline, as there is clear evidence of plasticity even in the final decades of life. In fact, the scaffolding theory of aging and cognition (STAC) integrates the ability of the maturing brain to adapt to age-dependent morphological changes to preserve cognitive function. STAC acknowledges that even if angiogenesis and neurogenesis are hindered, they remain present and compensate for white matter changes, decreased dendritic branching, altered synapses, and neurotransmitter changes. This new circuitry may not be as efficient, but does allow for continued neural functioning and resilience as illustrated in Fig. 10.2 [71]. This has been reinforced with rodent models and their demonstrated improvements in learning and memory, neurogenesis in the hippocampus, dendritic branching and synaptogenesis in the cerebral cortex, and basal ganglia with environmental enrichment [72]. In addition to these cellular examples of adaption/resilience in the aging nervous system, it is also clear that there are large-scale shifts in the neural networks and brain regions that give rise to cognitive task performance in older adults [26].


Fig. 10.2
A revised model of scaffolding theory of aging and cognition (STAC-R) is shown here. It encompasses both progressive dysfunction of the nervous system along with behavioral and biological compensatory mechanisms seen with aging (Reprinted from Reuter-Lorenz and Park [148])
However, not all neuroscientists are as optimistic about the preserved function of the aging brain. Mahncke identifies four core factors that precipitate the “downward spiral of degraded brain function in older adults”: reduced schedules of brain activity, noisy processing, weakened neuromodulatory control, and negative learning . Collectively, he states these processes cause brain plasticity to negatively impact functional pathways in an inevitable fashion. Therefore, this group poses that preventing or minimizing these changes is the best hope to preserve neurological function [73].
Though neural plasticity is still present with age, an examination of recovery from stress-induced neuronal atrophy in the prefrontal cortex of rodents established that aging impairs neural resilience and synaptic plasticity. Therefore, the advances that can be made with behavioral and biochemical interventions cannot completely counterbalance the impact of healthy aging [10, 74]. This area remains ripe for further research and continued efforts to promote regeneration and rejuvenation in the maturing brain have included physical and cognitive exercise, careful caloric restriction, young plasma administration, and stem cell use [75, 76]. Furthermore, it is unclear to what extent rodent models faithfully reflect the aging human brain, and therefore, it will be critical to evaluate where these therapeutic strategies are also effective in the aging human brain.
Pathologic Changes in the Central Nervous System
While there are numerous pathologies associated with the central nervous system, here we will focus on disease states that commonly occur in older adults.
Alzheimer’s Disease
The diagnosis of Alzheimer’s Disease (AD) is largely clinical; patients commonly follow an insidious course with progressive deficiencies in short-term memory (i.e., anterograde amnesia), word finding, spatial cognition, and executive function. A small fraction of AD cases display Mendelian inheritance patterns due to specific genetic mutations (and are thus termed familial AD), but the vast majority of AD cases are not caused by simple dominant or recessive acting genetic mutations, and thus occur sporadically. In either case, the diagnosis of AD requires the absence of another likely causes such as significant cerebrovascular disease or evidence of other dementias. This diagnosis can be further corroborated by MRI findings of hippocampal atrophy, CSF changes in amyloid-β and tau levels, neuroimaging evidence of brain amyloid-β and tau deposition, autopsy findings of neurofibrillay tangles and senile plaque deposition, and temporoparietal dysfunction. Patients with genetic mutations such as APO-E ε4 are also at increased risk of developing sporadic AD, and additional genetic variants that may contribute to the risk of developing “sporadic AD” are still being discovered [77, 78]. Acetylcholine is the neurotransmitter most influenced by the disease – with postmortem studies showing preferential nicotinic receptor loss [48], though changes in dopamine and serotonin have also been observed [79].
Due to the concern that the first pathological changes in AD occur decades earlier than behavioral or cognitive manifestations, there is a tremendous focus to identify features of preclinical AD to further direct therapeutic options. Additionally, due to concern that perioperative stress and anesthetic drugs may cause an accumulation of amyloid-β and worsening of tau pathology, understanding the pathophysiology of AD is immensely important [80].
Research has demonstrated that in addition to the heterogeneous myelin breakdown seen with aging there is a global myelin breakdown in AD due to extrinsic insults such as amyloid-β peptide [81]. An attempt to model the progression of the disease by the emergence and evolution of biomarkers was published in 2013. Jack et al. warned that amyloid-β reliably leads to plaque formation but will not always lead to clinical symptoms. This group proposed that even with biomarker evidence of AD there was individual variability in associated cognitive impairments. This variable manifestation of the disease was likely due to differing levels of cognitive reserve and concurrent medical conditions such as vascular disease [82].
Parkinson’s Disease
Parkinson’s Disease (PD) is a progressive neurodegenerative disorder caused by the death of dopamine neurons in the substantia nigra. This cell loss is accompanied by the formation of Lewy bodies, intracellular inclusions that contain the protein α-synuclein; mutations in α-synuclein have been implicated in Parkinson’s disease [83]. Similar to AD, the diagnosis of PD is made on clinical grounds, due to the presence of the cardinal motor symptoms including resting tremor, en bloc turning, bradykinesia, rigidity, and instability [84]. These motor manifestations trail other overt symptoms, such as olfactory dysfunction and sleep disorders [85, 86], so there has been a focused attempt to identify these early PD symptoms and genetic risk factors to help identify patients who might benefit from early symptomatic or even prophylactic treatment.
Currently, PD is often treated with the dopamine precursor levodopa, or L-DOPA. Unfortunately, patients often still develop nonmotor side effects including autonomic dysfunction, neuropsychiatric problems ranging from dementia to psychosis, and sleep disorders [87]. Levodopa offers symptomatic relief and delayed clinical progression, but it is not curative, and its benefits wane over time as the underlying disease process advances. Patients who have failed medical therapy are candidates for deep brain stimulation (DBS) . An impulse generator is connected to either the subthalamic nucleus or the internal segment of the globus pallidus which results in local release of adenosine and glutamate , increase in cerebral blood flow, and potentially proliferation of neural precursor cells. DBS also has chemical and electrical effects on dopaminergic pathways, and multiple clinical trials have shown clinical improvement in persistent motor symptoms [88]. Adverse effects include infection, hemorrhage, and unanticipated brain damage.
There are a host of genetic risk factors responsible for the development of PD, though environmental toxins and poisons can produce selective dopamine loss with a similar clinical profile [49, 73]. Regardless of etiology, patients with Parkinson’s disease are vulnerable to neurocognitive decline after exposure to general anesthesia in the setting of noncardiac surgery [89].
Lewy Body Dementia
Lewy Body Dementia (LBD) shares several neuropathological and neurochemical characteristics with PD and AD. LBD is characterized by the presence of cholinergic and dopaminergic dysfunction, lewy body pathology, cognitive impairment, and neuro-psychiatric symptoms. However key features that are relatively unique to LBD include vivid visual hallucinations, larger visuospatial deficits, and autonomic dysfunction [90].
AD and LBD may both demonstrate fluctuating cognitive deficits in additional to the clinical symptoms detailed above. However, morphological comparisons often help differentiate these two disease states. LBD is associated with cortical and subcortical atrophy but the temporal lobe and hippocampus remain preserved [91] (unlike AD, in which hippocampal and medial temporal lobe atrophy is often seen).
In comparison to PD, LBD patients typically develop cognitive symptoms before motor symptoms. LBD patients also cannot tolerate dopaminergic drugs as they often precipitate or exacerbate psychotic symptoms. As LBD patients cannot tolerate antipsychotics due to their autonomic dysfunction, it is imperative to avoid inappropriately treating LBD patients with antipsychotics [92].
Frontotemporal Dementia
Frontotemporal dementia (FTD) is a neurodegenerative disorder that is characterized by Pick bodies, intraneuronal inclusions, and generalized atrophy in the frontotemporal regions with associated emotional lability, poor social tact, and repetitive or compulsive behaviors. FTD onset typically occurs in middle age, though the age at diagnosis ranges from 35 to 75 years of age. Since many psychiatric disorders such as bipolar disorder and schizophrenia also commonly manifest in that age range and with similar symptoms as those seen in FTD, FTD is often misdiagnosed as a primary psychiatric disorder [93].
Additionally, it is also frequently misdiagnosed as early onset Alzheimer’s disease. One way to distinguish these disorders is to note that AD does not typically present with such social impropriety or impulsiveness. Furthermore, FTD is accompanied with progressive language dysfunction, and motor abnormalities such as muscle wasting and wasting. Objective testing, such as neuropsychiatric inventory scores, also demonstrate increased apathy, euphoria, and aberrant motor behavior in FTD and can help differentiate between these disease states [94].
This remains important when considering treatment options for patients with FTD. Unlike patients with Alzheimer’s, patients with FTD show no improvements and some undergo worsening of symptoms with acetylcholinesterase inhibitors such as donepezil [95]. SSRIs have been identified as a possible therapeutic avenue for the behavioral symptoms that accompany FTD [96].
Multiple Sclerosis
Multiple sclerosis (MS) is an autoimmune neurological condition defined by the presence of CNS plaques in at least two separate areas of the CNS associated with at least two different episodes of clinical symptoms that are at least 1 month apart [97]. These symptoms are a function of demyelination and associated inflammation. The clinical course of the disease often follows one of three pathways: relapsing and remitting, progressive, or a combination of the two. Though typically diagnosed in young adulthood or middle age, it is a disease that is still present in older adults. The cumulative effect of recurrent attacks can cause chronic inflammation with associated sustained sensory disturbances, ataxia, muscle weakness and spasms, visual disturbances, bladder dysfunction, fatigue, and neuropathic pain. Depression and anxiety are commonly found as well. A characteristic feature of multiple sclerosis is Uthoff’s phenomenon, a transient exacerbation of symptoms with an increase in temperature. Thus, vigilant monitoring of temperature during anesthesia is vital [98, 99].
Additional Pathologic Changes
It is difficult to isolate the specific disease process-associated changes in the central nervous system noted above from the age-dependent effects of common systemic diseases often seen in older adults. For example, hypertension has been shown to accelerate hippocampal shrinkage in a cumulative and progressive fashion [100]. Higher systemic pressures are also known to shift the autoregulatory curve for cerebral perfusion to the right, requiring relatively higher pressures for adequate cerebral blood flow. A prolonged history of hyperlipidemia, altered glucose homeostasis, and pro-inflammatory states may lead to accelerated aging or create alternative dysfunctional mechanisms in the central nervous system. It is therefore fundamental to understand the profound influence a patient’s overall health has on potentially exacerbating the specific disease-associated changes detailed above.
Peripheral Nervous System
Natural Changes
The peripheral nervous system (PNS) is also susceptible to progressive age-related changes. PNS neurons show a nonlinear atrophy pattern similar to that seen in the CNS: a selective decline in neuronal density and organization with loss of myelin integrity. Moreover, there is a reduced rate of axonal transport (neurotrophic factors, neurotransmitters, and receptors) and an increase in inflammatory markers (mast cells and macrophages) within the endoneurium. Collectively, these morphological changes are associated with a decrease in nerve conduction velocity that was initially observed in rodent models, and which is also seen in humans [101]. These findings likely also contribute to the decreased muscle strength, coordination, and proprioception commonly observed in older adults.
Loss of muscle mass and function with age are perpetuated by changes in the neuromuscular junction.
Histological and in vivo imaging demonstrate fewer synaptic vesicles and altered mitochondrial content in the aging neuromuscular junction [102]. Despite the decrease in synaptic vesicles, each vesicle appears to release a greater number of neurotransmitters. However, this increase in quantal size may be offset by increased neurotransmitter turnover and decreased postsynaptic end plate number and density [103, 104]. It is currently believed that oxidative stress and mitochondrial dysfunction may mediate some of these changes. The changes seen at the neuromuscular junction are summarized in Fig. 10.3 [105].


Fig. 10.3
Summarized are the functional and structural changes seen with aging in the neuromuscular junction (Reprinted from Gonzalez-Freire et al. [105])
Protective mechanisms help offset these effects; studies demonstrate that older peripheral nerves have lower energy requirements than younger peripheral nerves, which may protect these nerves against potential ischemia due to decreased vascular blood flow [106]. Neurogenesis also counteracts the deficits detailed above, but the degree and rate of regeneration diminishes with age. This is attributed to the impairments in both Wallerian degeneration (less mitogenic factor release from macrophages) and axonal regeneration (less robust response from Schwann cells) [107–109].
Natural Changes in the Autonomic Nervous System
The Autonomic Nervous System (ANS) is likewise affected by aging. The sympathetic nervous system has a more robust neurotransmitter presence in the elderly, particularly in the heart and skeletal muscle. This is partly due to an augmented release of norepinephrine and decreased clearance of norepinephrine and epinephrine [110, 111]. Additionally, older individuals demonstrate a concurrent decrease in alpha and beta receptor sensitivity, and decreased alpha-dependent intracellular responses. Together, these changes in adrenergic receptor expression result in a lower maximal heart rate and vasoconstriction [112, 113]. Therefore, despite increased catecholamine concentrations, physiologic responses to stress are considerably diminished [114].
While there are likely changes in the parasympathetic nervous system with age, there is limited research to appreciate such changes and their clinical outcomes. Given the increase in orthostatic hypotension with age, and the deleterious consequences of poor perfusion or syncope in advanced age, age-related changes in the carotid baroreflex have been studied by many groups. It appears that despite the reflex being present with age older individuals have a blunted and delayed response to hypotension compared to their younger cohorts [115, 116]. Though the mechanism behind these changes remains unresolved, it is likely a confluence of neural and mechanical impairments in the reflex arc as well as potential gender-mediated differences [117].
Natural Changes in the Enteric Nervous System
An often overlooked part of the nervous system is the enteric nervous system (ENS) , which is composed of epithelial cells, muscles, and neurons and regulates gastrointestinal function. The ENS is also known to undergo dynamic changes with aging through poorly elucidated mechanisms. Substantial age-associated neuronal loss (quoted as high as 40–60%) has been demonstrated in animal models, with a particular emphasis on cholinergic neuron loss. However, consistency regarding the degree of loss is lacking across animal species . It is believed that oxidative stress, free radical damage, decrease stores of neurotrophic factors, replicative senescence, and degenerative changes in the intestinal epithelial barrier are potential mechanisms for enteric neuron loss. While caloric restriction may help mollify such changes, neurogenesis in the ENS during adulthood has not been identified [118]. The clinical implications of ENS impairments seen in older patients should prompt anesthesiologists to consider adjusting aspiration prevention guidelines for older adults due to delayed motility and transit of intestinal contents, increased incidence of gastroesophageal reflux, and diminished oropharyngeal responses including the gag reflex [119].
Age-Dependent Pathological Changes in the Peripheral Nervous System
ALS
Amyotrophic lateral sclerosis (ALS) is a fatal neurodegenerative disorder defined by progressive motor dysfunction secondary to loss of upper and lower motor neurons. The etiology remains unknown though possible mechanisms include oxidative stress, mitochondrial dysfunction, glutamate-mediated neuronal excitotoxicity (perhaps leading to increased intracellular calcium homeostasis), and inflammatory stress. Familial ALS has been associated with mutations in the SOD1 gene; however like AD, the vast majority of ALS cases are not inherited in a Mendelian fashion, and thus can be considered “sporadic” [120]. Histological hallmarks seen in familial and sporadic ALS include astrocytic gliosis and intraneural inclusions in the affected neurons [121].
Sensation remains intact in ALS, as the disease affects only motor pathways, though it typically spares muscles involved in eye movement and the urinary sphincter. The progressive loss of motor neurons causes patients to experience fasciculations, cramps, spasticity, weakness, and muscle atrophy. Patient psyche is also affected, and patients show an increased incidence of dementia and depression. Curative therapy remains elusive, and patients inevitably face a steady decline in function and independence requiring discussions about ventilatory support and ultimately end-of-life care. Anesthesiologists often care for ALS patients undergoing tracheostomies and gastric tube placements.
Peripheral Neuropathies
Peripheral neuropathy in older adults most frequently occurs secondary to systemic events such as peripheral vascular disease and diabetes, though it can also be caused by malignancy, autoimmune disease, toxins (alcohol and drugs), nutritional deficiencies (particularly vitamin B12), medications (chemotherapeutic agents), and idiopathic causes [122]. Therefore, one must appreciate the complete medical history of a patient and the cumulative impact of these conditions when contextualizing neurological findings. Ascertaining the etiology of peripheral neuropathy in older adults is essential, for treatment modalities vary drastically and depend greatly on the mechanism of denervation.
Anesthetic Implications
Currently, more than one third of inpatient surgeries in the USA are performed in patients over age 65, and with the aging baby boomer generation this number will only increase. Thus, it is pivotal for anesthesiologists to learn the acute nuances in treating the geriatric population [123] (see Chap. 1). Anesthesia in elderly patients must be tailored to appreciate these age-dependent changes in the physiology of the human nervous system, and the increased incidence of nervous system pathology in older patients. As patients often cannot communicate during intraoperative management, our clinical judgment relies heavily on monitors that must be interpreted appropriately for older patients.
Even management of vital signs differ in the geriatric population, since older adults frequently do not adequately respond to stressors such as hypovolemia, hypothermia, hypoxia, and infection. Studies demonstrate that older adults have a lower maximal heart rate, require higher systolic pressures for adequate perfusion of vital organs, have impaired cardiovascular responses to hypotension, and exhibit impaired vasoconstriction during cold exposure [124, 125]. Given these attenuated physiologic responses and heightened activation of the sympathetic nervous system detailed earlier, one can conclude older individuals will have an unpredictable response to direct sympathomimetics with possible lower ceiling effects, highlighting the need for careful titration of vasopressors and inotropes.
Nonetheless not all reflexes are attenuated in older adults; dynamic cerebral autoregulation remains preserved with age despite impediments in cerebral blood flow and the baroreceptor reflex when exposed to orthostatic stress [31]. When studied in the context of volatile anesthetics, elderly patients require maintenance of mean arterial pressure and cerebral blood flow for preservation of dynamic autoregulation and tissue oxygenation [126]. This further emphasizes the importance of cardiovascular and respiratory intraoperative management to preserve neurological function in the geriatric population.
It is well accepted that the minimal alveolar concentration (MAC) for inhaled anesthetics declines by ~6% per decade after age 30 [127] (see Chap. 16).This is unsurprising as older patients are also more susceptible to other CNS-associated medications such as benzodiazepines, antidepressants, and antipsychotics [128] (see Chap. 17). Likely, this is due at least partly to altered pharmacokinetics, pharmacodynamics, and receptor sensitivity. Equally important is the notion that with “less wiring more firing” there are fewer functional myelinated tracts for anesthetics to act on. This would imply that anesthetic dosing is dependent upon white matter mass and functional neural fibers and not upon electrical activity which is largely preserved with age. However, as the correlation between anesthetic dosing and white matter mass and whole brain responses has not been studied, this remains a ripe area for research.
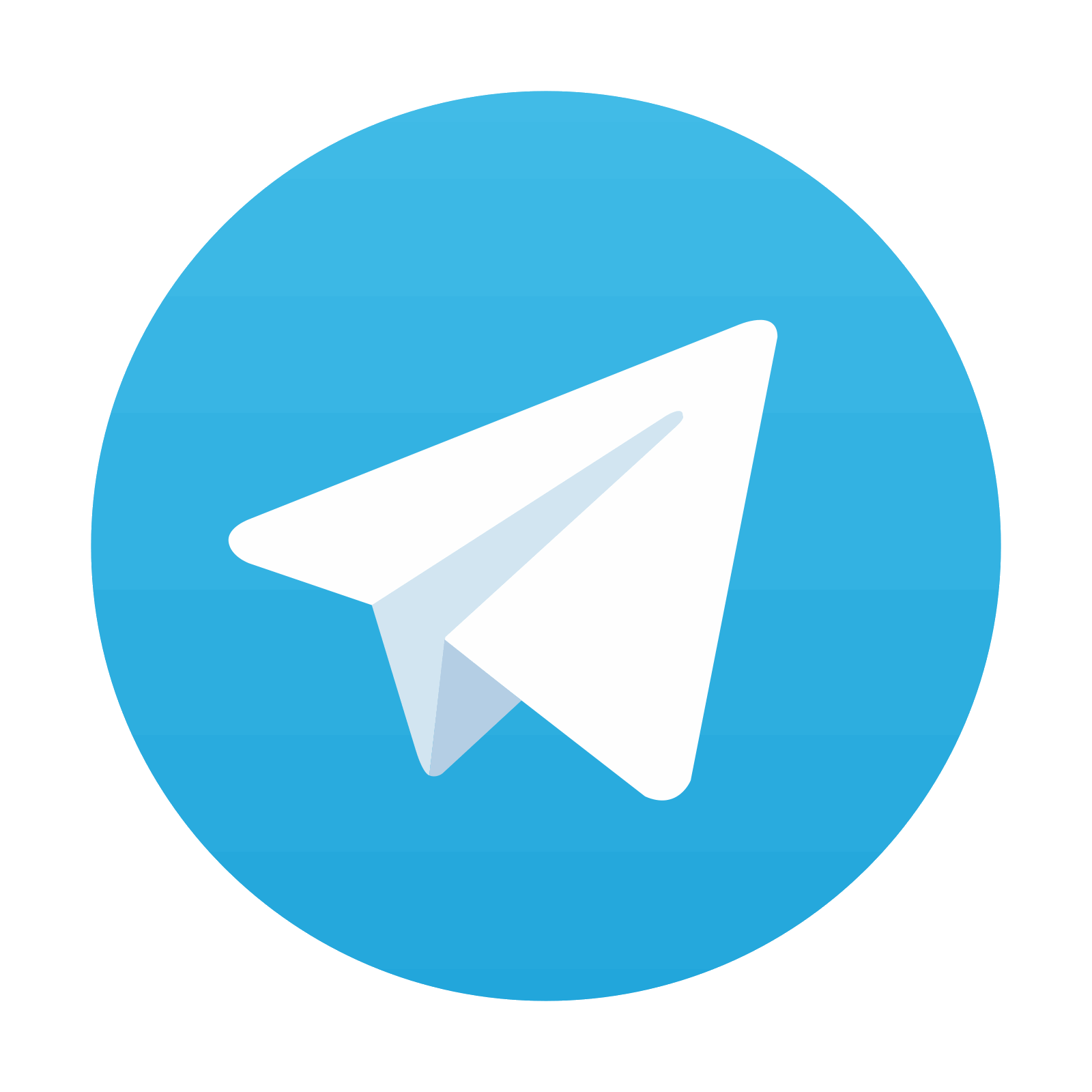
Stay updated, free articles. Join our Telegram channel
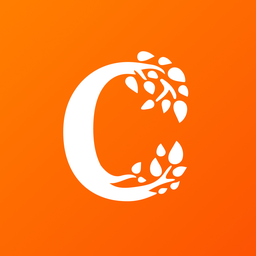
Full access? Get Clinical Tree
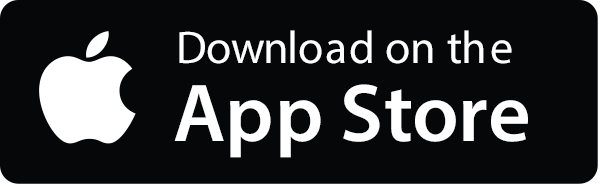
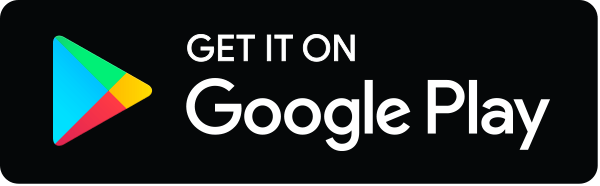