Peripheral arterial disease (PAD) caused by atherosclerotic occlusions that impair blood flow to the lower extremity is now recognized as a major health care problem. Despite the growing enthusiasm and improvements in both surgical and percutaneous techniques to improve blood flow to ischemic lower extremities in PAD,1,2 a sizeable portion of patients with PAD, and especially those with critical limb ischemia (CLI), cannot benefit from these procedures because of diffuse atherosclerotic disease, poor distal conduits, or comorbid conditions that make them poor revascularization candidates. Angiogenesis can be defined as the growth and proliferation of blood vessels from existing vascular structures, while therapeutic angiogenesis is an investigational method designed to employ the growth of new blood vessels to improve the vascular supply of ischemic tissues.3,4 The purpose of this chapter is to review the evolution of gene therapy as a method to induce therapeutic angiogenesis in patients with PAD. To that end, we will review the mechanisms of new blood vessel growth, gene therapy vectors and delivery techniques, and human clinical trials that have used this technology to date. Finally, we discuss the recent studies of novel gene therapy approaches to therapeutic angiogenesis.
In the adult organism, blood vessel number is under tight biologic control. New blood vessel formation, or neovascularization, is an extremely complex process that is tightly regulated, both temporally and spatially, and involves the production and interaction of numerous cytokines and signaling molecules. Neovascularization can be categorized into three distinct components: angiogenesis, arteriogenesis, and vasculogenesis (Figure 40-1). Although the term angiogenesis is frequently used to describe the process of new blood vessel growth in general, it specifically denotes the sprouting of new capillaries from existing vascular structures.5 These capillary networks consist of only endothelial cell tubes and lack additional stabilizing components in the vessel wall. Angiogenesis can significantly expand the capillary bed, but the growth of larger vessels is often required to produce a significant increase in total blood flow. Tissue hypoxia or ischemia is considered the most important stimulus for angiogenesis.
FIGURE 40-1.
Mechanisms of neovascularization in the adult. (A) Angiogenesis describes the sprouting of new capillaries from existing structures. Smooth muscle cells (SMCs) and other stabilizing components of the vessel wall are necessary to produce mature vessels. (B) Vasculogenesis refers to the recruitment of EPCs from the bone marrow to areas of neovascularization. These cells may become incorporated into nascent capillaries or stimulate vessel growth via the local release of proangiogenic factors. (C) Arteriogenesis, or collateral vessel growth, denotes the maturation of existing collateral conduits upon occlusion of a supply vessel (e.g., by a thrombus). Sheer stress and monocyte recruitment are thought to result in the local production of cytokines that drives arteriogenesis.
Figure reproduced with permission from Carmeliet P. Manipulating angiogenesis in medicine. J Intern Med. 2004;255:538-561, and Carmeliet P. Mechanisms of angiogenesis and arteriogenesis. Nat Med. 2000;6:389-95.

Arteriogenesis refers to the maturation of existing collateral conduits, or perhaps de novo growth of new vessels, frequently large enough to be visualized angiographically (greater than 130 μm in diameter). Because this process leads to the formation of arterial conduits, arteriogenesis can significantly increase distal blood flow.6,7 It is thought that local shear stress and inflammation are the primary processes that stimulate arteriogenesis.8,9
Once thought to occur only during embryonic development, vasculogenesis denotes the in situ formation of blood vessels from circulating endothelial progenitor cells (EPCs).10,11 EPCs are CD34+ stem cells of bone marrow origin that are thought to migrate to areas of neovascularization and fuse with other EPCs and capillaries, leading to the local expansion of the vascular network. Recent studies, however, call into question the exact origin of so-called EPCs12,13 and challenge the notion that these cells truly promote angiogenesis by incorporating into vessel walls.14 Despite these uncertainties, it appears likely that all three components of new blood vessel growth contribute to neovascularization in the adult organism.
Tissue hypoxia, inflammation, and mechanical forces such as shear stress are all thought to play critical roles in neovascularization. Of these, tissue hypoxia-induced angiogenesis is the best understood. Tissue hypoxia of the lower extremity occurs during exertion in patients with intermittent claudication (IC) and is constant in the setting of CLI. Hypoxia induces numerous processes at the local and systemic levels aimed at maintaining tissue homeostasis and viability. These protective mechanisms include local angiogenesis to enhance blood flow, erythropoesis to increase oxygen carrying capacity and delivery, and local alterations in metabolism to favor glycolysis for local energy production over oxidative metabolism.15 The hypoxia-inducible factor (HIF) system is an example of a “master genetic program” that regulates many of these processes in the tissue.
HIF-1 is a heterodimeric transcription factor composed of the HIF-1α and HIF-1β proteins that bind specifically to a hypoxia-responsive element (HRE) in more than 40 hypoxia-inducible genes.16 HIF-1β (also known as aryl hydrocarbon receptor nuclear transporter or ARNT) is a stable subunit that is constitutively expressed under most conditions. HIF-1α, in contrast, has a half-life of less than 5 minutes during normoxic conditions because of the ongoing degradation via the ubiquitin proteosome pathway. Immediately following translation, the HIF-1α protein is tagged for degradation by prolyl hydroxylase-containing enzymes that require oxygen as a cofactor.17 This pathway is impaired in the setting of hypoxia, which causes a rapid increase in the intracellular levels of HIF-1α and subsequent heterodimerization with HIF-1β to form the stable HIF-1 complex. Numerous genes induced by HIF-1 are directly involved in angiogenesis, including the vascular endothelial growth factor (VEGF) family of genes, the angiopoitins, matrix metalloproteinases (MMPs), and others.16 The most salient of these with regards to angiogenic growth factor therapy are the VEGF family of genes.
The VEGF family contains five closely related genes: VEGF-A, -B, -C, -D, and placental growth factor (PIGF).18 Of these, the progangiogenic effects of VEGF-A (hereafter referred to as VEGF) have been most extensively studied. In the adult human, the VEGF mRNA transcript undergoes alternative splicing that results in the expression and secretion of at least four functional isoforms that are named based on the number of amino acid residues in the mature protein (the murine orthologs are each shorter by one residue): VEGF121, VEGF165, VEGF189, and VEGF206.18,19 The larger splice variants (165, 189, and 206) include exons that code for heparin-binding sequences. Whereas VEGF121 is freely diffusible in the extracellular environment, VEGF165 (the most highly expressed isoform) is partially circulating and partially bound to heparan sulfate proteoglycans in the extracellular matrix. The largest isoforms (189 and 206) remain fixed in the extracellular matrix. Because both VEGF121 and VEGF165 can be delivered systemically as soluble, recombinant proteins or by various gene transfer techniques, initial therapeutic angiogenesis trials of VEGF tested the effects of these isoforms. However, numerous studies suggest that the larger heparin-bound VEGF isoforms are important to angiogenesis in vivo.20,21,22
VEGF exerts its angiogenic effects via binding to two closely related tyrosine kinase receptors, VEGFR-1 (Flt-1) and VEGFR-2 (KDR/Flk-1), which are located primarily on the surface on endothelial cells.18 VEGF is required for normal embryonic vascular development23,24 and is a potent inducer of endothelial cell mitogenesis, chemotaxis, and survival, all of which are necessary for angiogenesis in the adult organism.18 VEGF also stimulates recruitment of blood-borne inflammatory cells, such as macrophages and T-lymphocytes, which are vital to initiating and maintaining the process of neovascularization.25,26
Arteriogenesis describes the growth of functional collateral arteries from preexisting arterio-arteriolar anastomoses that occur as a result of arterial occlusion.27 Unlike angiogenesis, the primary stimulus for arteriogenesis is not likely to be hypoxia. The initial trigger for arteriogenesis is thought to be changes in wall stress or shear stress that occur within a collateral arteriole after an increase in blood flow. These forces cause local endothelial cell activation, which induces the expression of multiple genes involved in chemoattraction and cell–cell adhesion.28,29 As with angiogenesis, numerous cytokines have been implicated in arteriogenesis. The members of fibroblast growth factor (FGF) family are perhaps the best characterized arteriogenic growth factors, and like VEGF have been studied extensively in preclinical and human trials.
The FGFs are a family of more than 20 structurally related growth factors that exert their proangiogenic effects through interactions with numerous high-affinity tyrosine kinase receptors (FGFRs) on the surface of target cells. FGFs 1 (acidic FGF), 2 (basic FGF), 4, and 5 have the most pronounced angiogenic effects, and like VEGF are potent inducers of endothelial cell proliferation, migration, and activation.30 Unlike VEGF, which acts primarily on endothelial cells, FGFs are capable of stimulating numerous cell types involved in vessel formation and maturation. These include pericytes, fibroblasts, and myoblasts, which are essential for muscularization of nascent capillaries.31,32 Also unlike VEGF, whose activity is determined at the level of growth factor expression, the biologic effects of FGF are regulated predominantly by the amount of FGF receptor expression and activation in target tissues.33,34
The rationale for the use of exogenous growth factors in PAD is that supplementation with growth factors can overcome the endogenous deficit of cytokines and produce a therapeutic angiogenic response.35 It should be noted, however, that little direct evidence exists to support the notion that cytokine growth factors are truly deficient in ischemic tissues. Despite this controversy, exogenous cytokine growth factor delivery is an accepted method to induce therapeutic angiogenesis and has been widely utilized. Cytokines can be delivered by direct administration of recombinant human protein or via gene transfer into target tissues, which results in subsequent protein production by transfected cells. Each method of growth factor delivery has its advantages and disadvantages. For example, although the transfer of protein cannot technically be classified as gene therapy, early attempts at angiogenic growth factor therapy utilized the delivery of recombinant protein to ischemic tissues. The primary advantage of protein therapy is the ability to deliver a known quantity of growth factor, and thus construct precise pharmacokinetic and dose–response relationships.36 However, the limited tissue half-life of angiogenic proteins may be insufficient to produce the sustained activation necessary for a robust and complete angiogenic response.37 The advent of gene therapy, which results in the prolonged expression of the protein of interest by transfected cells, circumvents this limitation. Gene transfer methods can be roughly divided into two categories: nonviral and viral.
The most basic method of nonviral gene transfer is direct incubation with unmodified plasmid DNA, often called “naked” DNA. The use of naked DNA is simple and well-tolerated because of its low toxicity and immunogenicity,38 but there are several factors that limit the efficacy of this method. The small amount of naked DNA taken into a cell generally leads to low gene transfer efficiency,39 although naked plasmids have been reported to induce relatively high levels of gene expression in muscle tissue.40 Naked plasmid DNA is also rapidly degraded after intravenous injection, which necessitates direct delivery into tissue, usually by intramuscular injection. A clear advantage of this approach is the ability to administer repeated doses of DNA.
Numerous methods have been used to enhance transmission efficiency of plasmid DNA. One is the coupling of plasmid DNA to carrier molecules such as liposomes or polymer complexes, which facilitate transport of the DNA across cell membranes.41 Cell targeting with DNA–liposome complexes can be achieved by conjugating specific proteins to the complex, which results in the liposome preferentially entering cells with the appropriate cell surface receptors.42,43 The use of ultrasound energy and microbubble echocontrast agents has also been used to enhance transfer of naked DNA. This technique increases cell membrane permeability by producing transient small holes through which DNA is rapidly translocated, and has been shown to enhance transgene expression in cell culture and animal models.44,45,46 One advantage of plasmid and carrier molecule-based therapy is their relative ease of production, especially when compared to viral vectors.
Because of the relatively low gene transfer efficiency of plasmid-based techniques, numerous viral vectors have been used in therapeutic angiogenesis. Replication-defective adenovirus systems are the most commonly used viral vectors and are approximately 1000 times more efficient in transfecting cells than plasmid DNA.47 Adenoviruses can transfect both dividing and nondividing cells. The viruses enter the cells directly through the Coxsackie-adenovirus receptor (CAR)48 and through specific integrins.49 Once internalized, the adenovirus can escape from the endosome and release its transgene into the cytoplasm. The adenovirus remains extrachromosomal and provides transient expression of the transgene for 1 to 2 weeks.50 In human trials, adenoviral vectors have caused inflammatory reactions, transient fevers, increases in liver transaminases, and one highly publicized death.51 Despite this, the safety profile of adenoviral vectors is well established and their use in clinical trials has by and large been free of severe adverse events. Other limitations of adenoviral vector use include the lack of sustained gene expression (since the virus remains extrachromosomal), the antigenicity of viral proteins, and the lack of tissue specificity.38
Other viral vectors that have been studied for angiogenic gene therapy include adeno-associated viruses (AAVs), lentiviruses, and retroviruses, all of which integrate into the host genome and can induce long-lasting transgene expression.39,52 AAVs can efficiently transduce skeletal muscle, myocardium, and blood vessels53,54 and cause a diminished inflammatory response compared to adenovirus.55 However, the utility of vectors that induce long-term transgene expression in the treatment of PAD is unclear, especially since prolonged and unregulated expression of potent growth factors may cause unwanted and deleterious side-effects. Thus, the transient growth factor expression produced by extrachromosomal adenoviral or plasmid vectors may be ideal in the PAD setting.56
There are several routes of vector delivery that have been used in human angiogenesis trials. Plasmid DNA is rapidly degraded in the bloodstream and therefore is usually administered locally by direct intramuscular injection. Since systemic delivery of adenoviral vectors results in liver uptake and expression, local delivery via intramuscular injection is also preferred when viral vectors are used. In the setting of proximal arterial occlusion, which is often present in PAD, direct injection into tissues may be the only feasible delivery method. Angiogenic proteins can be delivered systemically into the bloodstream, although physical targeting via catheter-mediated intra-arterial delivery is generally preferred.57
The literature is filled with studies that detail the use of cytokine growth factors, especially VEGF and FGF, to induce therapeutic angiogenesis in preclinical models of PAD. A systematic review of those data is beyond the scope of this chapter. Instead, we will focus on published phase I and phase II human clinical trials of cytokine growth factor therapy for PAD (Table 40-1). In humans, VEGF165 has been used in one published case report, two phase I uncontrolled, nonrandomized trials, and two phase II randomized, double blind, placebo-controlled trials. VEGF121 has been tested in two phase I trials and one large phase II randomized, double blind, placebo-controlled trial. FGF-2 has been investigated in one phase I and one large phase II trial, while one human phase I study with FGF-1 has been published. The human trials reviewed below can be described as the first “phase” of studies that examined the role of gene therapy in PAD. A second, more recent phase of human trials (both completed and ongoing) using novel cytokine growth factors and gene therapy constructs will be discussed later.
Published Phase I and Phase II Human Clinical Trials of Cytokine Growth Factor Therapy for PAD
Author/Name of Trial | Disease | Treatment | N | Comments | |
---|---|---|---|---|---|
Phase I | Baumgartner et al.60 | CLI | Intramuscular phVEGF165 | 9 | Improvement in perfusion by angiography and MRA |
Shyu et al.62 | CLI | Intramuscular phVEGF165 | 21 | Improvement in ABI and perfusion by MRA | |
Rajagopalan, et al.65 | IC | Intramuscular AdVEGF121 | 15 | Trend toward improvement in ABI and peak walking time | |
Mohler et al.66 | CLI | Intramuscular AdVEGF121 | 13 | Demonstrated safety | |
Lazarous et al.76 | IC | Intra-arterial FGF-2 protein | 19 | Demonstrated safety | |
Comerota et al.77 | CLI | Intramuscular FGF-1 plasmid | 51 | Improvement in symptoms, ABI, and ulcer healing | |
Phase II | Mäkinen et al.63 | IC and CLI | Intra-arterial VEGF165 via plasmid and adenoviral vectors | 54 | Delivered VEGF165 post-angioplasty; demonstrated increased vascularity |
Kusamanto et al.64 | CLI | Intramuscular phVEGF165 | 54 | Diabetic patients; improvements in wound healing and hemodynamics | |
TRAFFIC78 | IC | Intra-arterial FGF-2 protein | 190 | Improvement in peak walking time | |
RAVE67 | IC | Intramuscular AdVEGF121 | 105 | No difference in primary or secondary end points |
In 1996, Isner et al.58 published the first case report that described the use of gene transfer to treat PAD in a human subject. In this pioneering case, a 71-year-old woman patient with diabetes suffering from toe gangrene caused by multiple atherosclerotic arterial occlusions was treated with site-specific gene transfer of plasmid DNA encoding VEGF165 (phVEGF165). This plasmid consisted of a eukaryotic PUC 118 expression vector into which cDNA encoding VEGF165 has been inserted, and contains a cytomegalovirus promoter/enhancer to drive production. Gene transfer was achieved by the use of a hydrogel-coated balloon angioplasty catheter system that the same investigators had successfully tested in animal models.59 A sterile pipette was used to apply 2000 μg of plasmid DNA to the external hydrogel coating of the balloon. Under fluoroscopic guidance, this balloon catheter was inflated in the distal popliteal artery, proximal to occlusions of the peroneal, anterior tibial, and posterior tibial arteries.
Digital subtraction angiography (DSA) 4 and 12 weeks after gene transfer revealed increased collateral vessels in the treated limb at the knee, midtibial, and ankle levels (Figure 40-2). The investigators also noted increased intra-arterial Doppler flow measurements after gene transfer. In addition, magnetic resonance angiography at 4 and 12 weeks showed improved flow distal to the preexisting arterial occlusions. Approximately 7 days after gene transfer, the patient developed edema of the treated limb and three spider angiomas were noted over the medial and dorsal forefoot. The edema subsided within 4 weeks of treatment, and after excision of one angioma, the remaining two regressed by week 8 posttreatment. The investigators attributed all these findings to successful gene transfer of VEGF and subsequent gene expression in ischemic tissues. Despite an apparent improvement in this patient’s blood flow, she eventually required a below-the-knee amputation of the treated limb. This landmark study not only demonstrated that gene transfer with VEGF could promote angiogenesis in patients with PAD, but also highlighted the potential deleterious effects of VEGF gene transfer, specifically edema resulting from new blood vessel growth or enhanced vascular permeability.
FIGURE 40-2.
Selective digital subtraction angiography of the lower leg immediately before (left) and 4 weeks after (right) phVEGF165 gene transfer. New collateral vessels are visible.
Reproduced with permission from Isner JM, Pieczek A, Schainfeld R, et al. Clinical evidence of angiogenesis after arterial gene transfer of phVEGF165 in patient with ischaemic limb. Lancet. 1996;348(9024):370–374.

Baumgartner et al.60 Approximately 14 months after Isner’s initial report, the same group published a phase I nonrandomized human trial that used phVEGF165 in patients with chronic CLI. In this study, a total of nine patients (10 limbs) with nonhealing ulcers (7/10 limbs) and/or ischemic rest pain (10/10) who were not revascularization candidates were treated with intramuscular injection of phVEGF165. Hemodynamic criteria included a rest ankle-branchial index (ABI) <0.6 and/or toe brachial index (TBI) <0.3 in the affected limb on two consecutive examinations at least 1 week apart. Each patient received a total of 2000 μg of plasmid DNA (4 aliquots of 500 μg each) by direct intramuscular injection into the ischemic limb. Injection sites were chosen arbitrarily based on available muscle mass and sites were both above and below the knee. Patients received a second 2000 μg dose 4 weeks after the initial injections, and all injections were well tolerated. There was no evidence of tumor development or worsening retinopathy during the follow-up period of the study (the average length of follow-up was 6 ± 3 months).
In this report, the investigators provided what was felt to be direct and indirect evidence of successful gene transfer. Serum (not plasma) levels of VEGF (determined by enzyme-linked immunosorbent assay (ELISA)) peaked 1 to 3 weeks after gene transfer in the seven of nine patients from whom blood was collected, which demonstrated transgene expression at the protein level. Six of the nine patients developed clinically evident edema after gene transfer, and the edema was bilateral (although more severe in the treated limb) in two patients. Immunohistochemical staining of tissue obtained from one amputee 10 weeks after gene therapy treatment revealed proliferating endothelial cells, which is rare in normal arteries. Polymerase chain reaction (PCR) performed on the same tissue revealed DNA fragments unique to phVEGF165 in several tissue samples both near and remote from the sites of injection. In addition, Southern blot analysis of tissue obtained from two other treated amputees (8 and 10 weeks after treatment) confirmed the presence of DNAfragments unique to phVEGF165.
The authors reported several striking physiologic benefits of phVEGF165 treatment. DSA demonstrated newly visible collateral vessels at the knee, calf, and ankle levels in 7 of 10 treated limbs, and follow-up angiograms did not reveal evidence of collateral regression. Serial magnetic resonance angiograms of the ischemic limbs revealed qualitative evidence of improved distal flow in eight limbs. Absolute systolic ankle or toe pressure increased in eight limbs and was unchanged in one limb, and exercise performance improved in all five patients who were able to perform graded treadmill exercise. All patients also experienced a statistically significant increase in pain-free walking time (2.5 ± 1.1 minutes before gene therapy versus 3.8 ± 1.5 minutes after gene therapy), which was measured an average of 13 weeks after treatment. In addition, the frequency of ischemic rest pain, defined as nights with pain per week, decreased significantly (5.9 ± 2.1 nights/week at baseline versus 1.5 ± 2.8 nights/week) after therapy. Nine of ten treated limbs had an improvement in limb status based on the Rutherford criteria,61 with moderate improvement reported in five limbs. The investigators cautiously concluded that this study supported the strategy of intramuscular gene therapy with VEGF165 to induce therapeutic angiogenesis in selected patients with PAD.
Shyu et al.62 A second study of phVEGF165 in patients with chronic CLI was performed by Shyu et al.62 from Shin Kong Wu Ho-Su Memorial Hospital in Taipei, Tiawan. Patient selection and inclusion were similar to the study by Baumgartner et al.,60 and patients were treated with intramuscular (IM) injections of the VEGF165 plasmid in the same manner. This study sought to establish the minimal effective dose of phVEGF165 in a Chinese patient population. Gene transfer was performed in 24 limbs of 21 patients with rest pain, some of which also had nonhealing ulcers (n = 16). Doses ranging from 400 to 2000 μg were given at the beginning of the study, and the same dose was given 4 weeks later. A total of five patients received an additional 2000 μg “booster” injection 2 to 3 months after the second injection.
Mean plasma levels of VEGF increased significantly 2 weeks after the initial treatment, but the authors were unable to demonstrate a statistically significant dose–response curve. Transient edema occurred in 6/24 limbs, but only in patients treated with 1600 or 2000 μg injections. Rest pain resolved completely in 12 limbs and improved significantly in 8 limbs, and 12 of 16 limbs with nonhealing wounds had improved tissue integrity. Treatment failure was more frequent in patients treated with lower doses of plasmid. The mean ankle-brachial blood pressure index increased significantly from 0.58 ± 0.24 before treatment to 0.72 ± 0.28 (P < 0.001) 4 weeks after completing two injections. The increase in ABI in patients receiving 1600 μg of plasmid was significantly higher than patients treated with 400 or 800 μg of phVEGF165, but there was no apparent benefit seen with the 2000 μg dose. The mean magnetic resonance angiographic score also increased after treatment. Compared to the study by Baumgartner et al.,60 Shyu et al.62 noted a lower complication rate (predominantly due to less lower extremity edema) and a higher failure rate, which the authors attributed to the lower treatment doses of plasmid. The investigators concluded benefits of treatment with phVEGF165 required at least two 1200 μg injections.
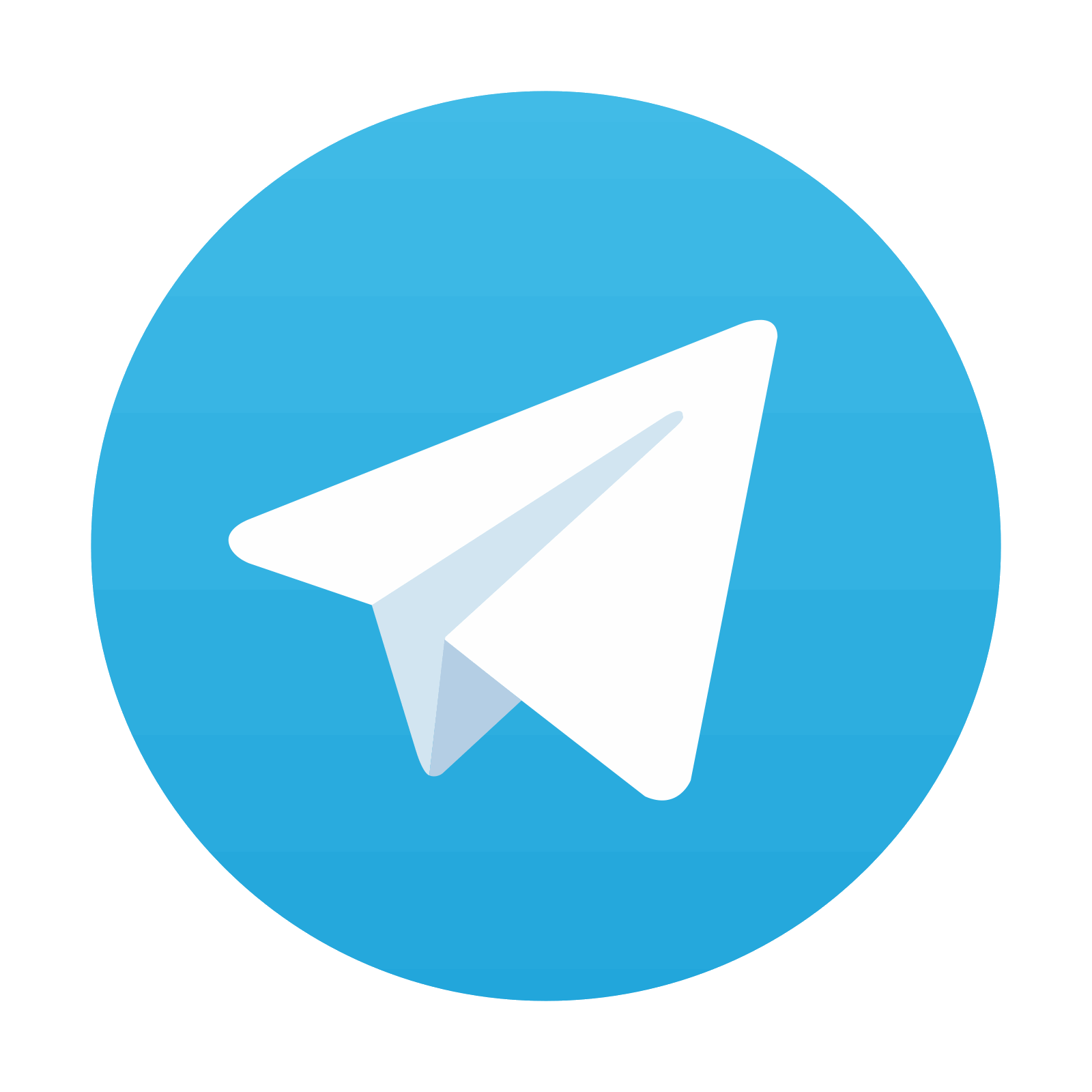
Stay updated, free articles. Join our Telegram channel
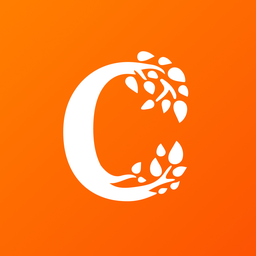
Full access? Get Clinical Tree
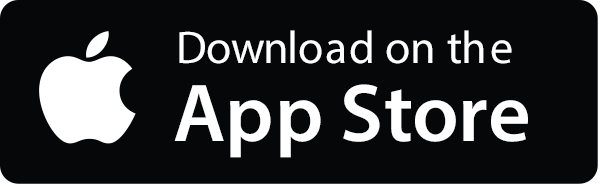
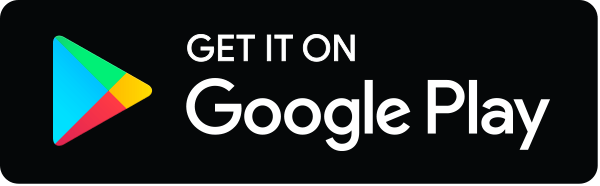