Gene Therapy
Elizabeth G. Nabel
Gene transfer is the introduction and expression of recombinant genes in mammalian cells. The human gene therapy field is young, having its origins in the mid-1980s. Major advances in the past 25 years have been made in defining scientific principles, developing animal models, and initiating human trials. Gene transfer has created genetic models of human disease, which have defined the pathophysiology of cardiovascular diseases. The concept of using a recombinant gene, rather than a drug, to treat a cardiovascular disease is relatively straightforward. However, the technical difficulties of selecting the appropriate gene and expressing it in a sufficient number of cells to achieve the appropriate biologic effect have been major challenges. In this chapter, gene transfer and cardiovascular disease are reviewed. The principles of gene therapy are defined. Viral and nonviral vectors used for cardiovascular gene transfer are reviewed. Animal models of cardiovascular disease based on gene transfer approaches are discussed. Finally, prospects for gene therapy of cardiovascular diseases are critically analyzed.
Glossary
Antisense RNA
A single strand of RNA that is complementary to RNA sense strand. Binding of antisense to sense strand creates a double-stranded RNA hybrid that is not translated into protein.
Coding region
The portion of the gene or messenger RNA (mRNA) that contains the base pair sequences that are translated into protein.
Complementary DNA (cDNA)
A single-stranded DNA sequence that is synthesized from the RNA strand by reverse transcriptase and is complementary to an mRNA coding region.
Electroporation
A method to transfect cells that employs an electrical field to affect the entry of DNA into cells.
Episomes
Genetic sequences that exist in stable form separate from the chromosome.
Exons
The segments of a gene that remain after splicing of the primary RNA transcript and make up mature mRNA, which in turn encodes protein.
Expression, stable
Expression of a gene that has been transfected into cells and is maintained long term in the target cell.
Expression, transient
Expression of a gene that has been transfected into target cells and is maintained short term in the cell.
Homologous
Common structural features found in two separate genes, which suggest a common ancestor gene.
Interfering RNA
Short 21 to 23 nucleotide interfering RNAs mediate degradation of target mRNA through incorporation into the target RNA and cleavage.
Intron
Noncoding gene sequences that are transcribed into the primary nuclear RNA transcript but excised during RNA splicing.
Oligonucleotides
A short polymer of DNA that is synthesized to perform studies of gene expression and function.
Plasmids
Naturally occurring circular DNA molecules that replicate in bacteria, which can be modified for use as vectors.
Promoter
The region of a gene that binds RNA polymerase and initiates gene transcription. Promoters can be constitutive-active in all cell types, cell-specific-active only in a specific cell type, or inducible switched on or off.
Recombination
The joining together of different segments of identical DNA sequence into a single continuous piece of nucleic acid sequence.
Reporter gene
A gene that is employed to assess the relative activity of a promoter or to encode a protein that is normally not found in the target cell, thereby allowing assessment of the promoter or gene expression in a target cell.
Reverse transcriptase
An enzyme that catalyzes the synthesis of DNA from an RNA template.
Sense strand
The single strand of DNA that gives rise to the RNA from which protein can be translated.
Transcription
The synthesis of mRNA from a DNA template by RNA polymerase.
Transfection
A technique that is used to transfer naked DNA to the inside of the cell, resulting in the expression of the gene in the target cell.
Translation
The synthesis of protein from the mRNA template by ribosomes.
Vector
A genetic carrier used to deliver exogenous DNA fragments into a host cell.
Historical Perspective
The gene therapy field has its origin in molecular biology and recombinant DNA technology. DNA was first discovered in 1944 as the genetic material in bacterial cells (1). Watson and Crick determined the double helical structure of DNA in 1953 (2). Leder and Nirenberg elucidated the genetic code by 1964 (3), and the first recombinant DNA molecules were synthesized using molecular biology techniques by 1972 (4). The scientific basis for gene therapy was developed in the 1970s, based on knowledge of the mechanisms of cell transformation by tumor viruses. The principles of gene transfer are based on the entry of foreign recombinant DNA into a host cell. Cells are resistant to the uptake of foreign genes, and viruses have adapted methods to gain entry into the cell. Early gene transfer methods adapted viruses (modified to be replication incompetent) to carry recombinant genes into cells. Basic investigations of tumor viruses, particularly retroviruses, laid the scientific foundation for the development of gene therapy in the 1980s. In the early 1990s, investigators used their knowledge of viral vectors and gene delivery to develop animal models of human disease (5). These experimental models evolved into the development of human gene therapy protocols in the United States. The first approved trial of gene transfer in humans was performed in May 1989 (6). A human gene therapy trial was initiated in 1990 for adenosine deaminase deficiency, which used ex vivo delivery of retroviral vectors encoding the human ADA gene into lymphocytes (7). Since this time, approximately 150 phase I–II clinical gene therapy trials have been performed in the United States, but no gene therapy treatments have been approved for clinical use by the Food and Drug Administration (FDA).
Principles and Concepts of Gene Transfer
Gene therapy is a set of approaches to the treatment of human disease based on the transfer of genetic material into an individual. Gene delivery can be achieved either by direct introduction of vector or DNA to blood or tissues, or indirectly through the modification of cells that have been transduced to carry the foreign DNA. Gene therapy treats a disease in an individual by the administration of DNA rather than a drug. Somatic cells, not germ cells, are the target of these efforts. Therefore, gene therapy affects only individuals and not their offspring.
An initial step in the delivery of genes to individuals is the construction of gene transfer vectors (Fig. 97.1). A vector carries the recombinant gene (or DNA or RNA) into cells within a tissue. Vectors are used to transfer genes into cells because most cells are resistant to the uptake of foreign DNA. Vectors penetrate host cells with varying efficiency, depending on the cell type and the vector. Viral vectors include retroviral vectors, lentiviral vectors, adenoviral vectors, and adeno-associated vectors. Nonviral vectors include plasmid DNA, cationic liposomes, and interfering RNA.
After construction of a vector, the vector is generally tested in the laboratory in cultured target cells. If the gene is adequately expressed in these target cells, then animal models are developed in vivo. Gene delivery can be achieved by two methods: ex vivo and in vivo approaches. Ex vivo gene transfer involves the removal of autologous cells from the individual, genetically modifying or transducing the cells in culture, and returning the genetically modified autologous cells to the individual (8). Ex vivo gene transfer permits the introduction of recombinant genetic material into a particular cell type (e.g., a smooth muscle cell) and analysis of gene expression within that cell type (9). Ex vivo gene transfer, however, is a cumbersome and costly procedure, requiring the establishment and modification of cells in the laboratory. In vivo gene transfer, on the other hand, is a relatively straightforward approach by which the vector harboring the foreign DNA is directly inserted into host cells in vivo. This might be achieved by direct injection (10) or catheter-mediated gene delivery (11). After in vivo gene transfer, recombinant genes are generally expressed in multiple cell types.
The fate of recombinant DNA, once inside host cells, has been difficult to regulate and control. Considerable efforts have been devoted to developing methods for retaining the correct function of the gene and for regulating the gene so that the physiologic effects of gene expression can be evaluated.
As the gene therapy field has grown and seasoned, several important concepts have emerged. First, genetic defects contribute to the pathophysiology of many human diseases. In addition to monogenic or single gene disorders, many acquired diseases are characterized by normal genetic structure but abnormal expression of genes. For example, in atherosclerotic arteries, normal repair of an injured artery leads to abnormal expression of cytokine and growth factor genes (12). Many human diseases, both inherited and acquired, are characterized by genetic mutations that result in abnormal gene expression and pathophysiology.
Second, the fields of genetics and gene therapy, in the past, have developed in parallel, but there is increasing recognition of the interdependence of the two areas. The human genome has been mapped (13). The fields of genomics and proteomics
are emerging. Studies of gene expression and protein mapping will influence the diagnosis and treatment of cardiovascular diseases. Genomics, proteomics, and gene therapy complement one another and must be developed together.
are emerging. Studies of gene expression and protein mapping will influence the diagnosis and treatment of cardiovascular diseases. Genomics, proteomics, and gene therapy complement one another and must be developed together.
Vectors for Gene Transfer
Gene transfer vectors have relied on normal mechanisms of viral infection of human cells. For example, viruses have evolved mechanisms for receptor-mediated entry into human cells. Once within, a cell may reside latent for long periods. On viral activation by cytokines or other host genes, active viral replication occurs. In a similar manner, researchers have modified viruses to become vectors that carry a recombinant gene into a cell but cannot replicate within the cell. The recombinant gene resides within the nucleus, where it either integrates into host genome or undergoes episomal replication.
Viral and nonviral vectors have been developed and evaluated for cardiovascular gene therapy (Table 97.1). Viral vectors (retroviruses, lentiviruses, adenoviruses, adeno-associated viruses) have been the major methods for delivery of genes into cells. In response to concerns about the safety of viral vectors, nonviral vectors (plasmid DNA, DNA liposome complexes, interfering RNA) have also been developed. Each vector has advantages and disadvantages, which are dependent on the particular application, including cell type and organ system. Selection of a vector should consider the following factors. First, specificity to target tissues is critical. The vector should achieve local gene expression within an organ without systemic shedding and widespread toxicity. The vector should be delivered to host cells in sufficient quantity to result in high-level gene expression. Expression of the gene should persist for a sufficient period of time to treat the disease. Gene expression preferably is regulated through tissue-specific promoters or inducible or repressible promoters, or both. Finally, regulatory concerns about possible vector and gene toxicity should be kept in mind.
TABLE 97.1 Somatic Gene Transfer Vectors | ||||||||||||||||||||||||||||||||||||||||||||||||||||
---|---|---|---|---|---|---|---|---|---|---|---|---|---|---|---|---|---|---|---|---|---|---|---|---|---|---|---|---|---|---|---|---|---|---|---|---|---|---|---|---|---|---|---|---|---|---|---|---|---|---|---|---|
|
Several approaches have been developed to achieve site-specific gene expression. These include ligand-directed vectors, targeted gene expression using cell-specific promoters, catheter-mediated gene delivery, and direct injection. In the cardiovascular system, catheter-mediated gene delivery has been used most often to achieve site-specific gene expression within focal segments of blood vessels (11). Direct injection may be a feasible approach for gene delivery into the myocardium (14). Cell-specific gene expression may be desirable for selected approaches to vascular gene transfer, such as inhibition of
inflammatory cells or smooth muscle cell proliferation. Several laboratories have identified smooth muscle and endothelial cell-specific promoters, which have useful features for gene transfer vectors (15,16).
inflammatory cells or smooth muscle cell proliferation. Several laboratories have identified smooth muscle and endothelial cell-specific promoters, which have useful features for gene transfer vectors (15,16).
Viral Vectors
Retroviral Vectors
Retroviral vectors were the first viral vectors developed for gene transfer. These vectors promote efficient gene transfer and expression in cultured primary cells from humans and other animals. Retroviral vectors are replication defective, meaning that they do not encode any viral proteins and do not replicate within the host cell. The process of gene transfer and expression with retroviral vectors is called transduction, as opposed to infection, to differentiate this process from viral infection, which implies further viral replication and spread.
Although retroviral vectors were initially attractive, their use has waned. Disadvantages of these vectors include low titer production. Transduction is limited to dividing cells, thus lowering the number of applications to human diseases. Gene expression after retroviral transduction is difficult to control and stabilize. The most important, unacceptable side effect is the random integration of retroviral sequences into human chromosomes, which can lead to cell transformation and malignancy, as has been reported recently in a human trial for ADA deficiency (17,18).
Applications of retroviral vectors include marking studies in which autologous cells are removed from an individual, transduced to express a reporter or marker gene in vitro using a retroviral vector, and given intravenously back to the individual. These vectors have been employed for other ex vivo biological experiments, but their use in vivo has now been restricted because of reports of leukemias complicating retroviral gene therapy for ADA deficiency (17,18).
A pseudotyped retrovirus is a retrovirus that incorporates the envelope protein of a second virus (19). The host range of the pseudotype is that of the virus donating the envelope protein. The envelope glycoprotein (G) of the vesicular stomatitis virus (VSV), a member of the rhabdovirus family, can be efficiently incorporated into a retroviral particle. VSV-G pseudotype retroviral vectors have an increased host range, because VSV infects cells ranging from insects to primates, unlike the amphotropic virus. The VSV-G pseudotype vectors are also able to infect mammalian cells more efficiently than conventional amphotropic vectors. VSV-G pseudotyped retroviral vectors can be concentrated to titers greater than 109 CFU per mL. Therefore, VSV-G pseudotype retroviral vectors have gained favor because they extend the use of retroviral vectors for stable gene transfer into species not previously accessible to retroviral infection. Also, they allow more efficient gene transfer into mammalian cells for possible treatment of human diseases. The production of pseudotyped retroviral vectors is similar to retroviral vectors (18). Applications of pseudotype retroviral vectors are not established, but are similar to retroviruses.
Lentiviral Vectors
More recently, modified forms of another retrovirus, the human immunodeficiency virus (HIV), a lentivirus, have been described. Such lentiviral vectors have the ability to infect nondividing cells. These vectors have been used primarily for biological experiments examining gene expression within cells in tissue culture in noncardiovascular systems. The applicability of lentiviral vectors for cardiovascular gene transfer and therapy has not been adequately explored.
Adenoviral Vectors
Viral vectors based on human adenoviruses have shown considerable promise for in vivo gene delivery compared with retroviruses, because they can transfer recombinant genes efficiently into a wide variety of dividing and nondividing cells. Adenoviral vectors are also attractive because large amounts of highly purified recombinant virus, 1012 particles per mL, can be produced from expansion of viral plaques. The human adenovirus is a double-stranded DNA virus with a 36-kb linear genome that contains four early transcription units (E1 to E4), which are active at early time points after infection (3 to 12 hours), and five late transcription units (L1 to L5), which are active after 12 hours and produce the structural genes of the virus.
Adenoviral vectors are propagated in packaging cell lines, such as 293, which supply E1 function in trans. The final vector, however, is replication defective. Adenoviral vectors are constructed using a plasmid that contains the recombinant gene of interest and flanking adenoviral sequences. This adenoviral transfer plasmid is cotransfected with an adenoviral backbone into 293 cells. Recombination occurs in the region of overlap, in the E1 region between the restricted viral DNA or viral backbone and the plasmid. Viral plaques are produced, which then can be expanded. After further rounds of plaque purification, a stock of the adenoviral vectors is produced.
Transduction of host cells by adenoviral vectors follows the principles of adenoviral infection of epithelial cells. When the viral genome enters the cell, the early immediate genes (E1) are expressed. They activate other important regulatory early genes, E2 to E4. In the second phase of replication, late genes, L1 to L5, are expressed, leading to the production of more virus and cell death. The adenovirus has been engineered to be replication defective by deleting the early adenovirus genes that activate other viral genes. In the absence of these regulatory genes, the virus should not replicate in vivo, and the late viral genes should remain dormant. The first-generation adenoviral vectors have deletion of E1 genes and partial deletion of E3 genes, which renders them replication defective.
From extensive experience with first-generation adenoviral vectors, several themes have emerged. Inflammation occurs with first-generation adenoviral vectors in a dose-dependent manner at the site of gene transfer, but there are differences among species and tissues within species in the quality and quantity of the inflammation. A consistent finding is that the expression of genes after adenoviral transduction is transient. Attempts to restore expression by the administration of a second dose using the same serotype of the vector are usually not successful. These limitations have tempered the initial enthusiasm for first-generation adenoviral vectors. To overcome these immune problems, second-generation adenoviral vectors have been engineered to minimize the expression of viral antigens. Applications of adenoviral vectors include localized in vivo treatments, such as vascular diseases (20,21,22) and cystic fibrosis (23).
Adeno-Associated Viral Vectors
Adeno-associated viral (AAV) vectors have been explored for gene transfer. Recombinant AAV (rAAV) vectors are based on nonpathogenic human parvovirus, an adeno-associated virus. rAAV have two important advantages: their physical stability, which allows in vivo administration, and their ability to maintain stable gene expression.
rAAV can infect many cell types, including quiescent cells. Wild-type AAV infection results in integration into host chromosomes, where it exists as a stable provirus. Wild-type AAV has preferences for insertion into certain chromosomal locations. If latently infected AAV-infected cells are subsequently infected with adenovirus, the AAV genome is rescued or excised from the chromosome, replicated, and packaged to
produce progeny virions. This is the principle behind construction of rAAV vectors. The production of rAAV involves cotransfection of a rAAV-vector plasmid and the AAV-helper plasmid into 293 cells. These cells are subsequently infected with adenovirus to produce progeny virions. The rAAV virions are then separated from the adenovirus on cesium chloride gradients. rAAV stocks with titers as high as 1010 particles can be produced.
produce progeny virions. This is the principle behind construction of rAAV vectors. The production of rAAV involves cotransfection of a rAAV-vector plasmid and the AAV-helper plasmid into 293 cells. These cells are subsequently infected with adenovirus to produce progeny virions. The rAAV virions are then separated from the adenovirus on cesium chloride gradients. rAAV stocks with titers as high as 1010 particles can be produced.
Transduction performed in vivo using rAAV factors has shown long-term gene expression; furthermore, the level of gene expression does not decrease substantially over time. Studies are in progress to evaluate the application of this vector to the vasculature and myocardium. There has been greater initial success in the myocardium (24) and limited to no success in the vasculature (25). The major advantage of rAAV is integration at specific sites in host cell genome as well as persistent expression. Major disadvantages of rAAV include the lack of helper cell lines, size limitation of the recombinant genome that can be packaged (4,681 bases), and a requirement for replicating adenovirus to grow. Eventual applications of rAAV vectors may be similar to adenovirus, including localized in vivo treatments.
Nonviral Vectors
Nonviral vectors offer safety advantages for a number of applications to human gene therapy. Nonviral vectors currently in development include plasmid DNA, DNA liposome complexes, and interfering RNA.
Plasmid DNA
Plasmid DNA can be delivered to cells, under certain conditions, in the absence of a vector shuttle. The use of plasmid DNA alone offers several advantages, including ease of preparation. There are no size constraints for insertion of the transgene into the plasmid backbone. Experience with laboratory preparation and use of plasmid DNA in human trials has demonstrated considerable safety and lack of toxicity (26). There are no viral genes within the plasmid backbone. Expression of plasmid DNA within host cells does not require integration into host genome. Disadvantages of plasmid DNA include inefficient entry and uptake into cells, as well as limited persistence and a lack of stability. The major applications of plasmid DNA include topical or mechanical applications, or both, including catheter-mediated gene delivery, direct injection into tumors, and vaccination by direct injection into skeletal muscle. Several human gene therapy trials have used direct injection of DNA to treat cancer (26), as well as catheter-mediated gene delivery of plasmid DNA to the vasculature (27).
DNA Liposome Complexes
Cationic liposomes are positively charged lipid molecules that complex with negatively charged DNA and facilitate entry into cells. The mechanisms by which cell entry occurs are not well worked out, but receptor-mediated endocytosis or fusion with cell membranes is most likely. After entry into the cell, the lipid DNA complex is engulfed by lysosomes. Dissociation of lipid from DNA occurs with escape of plasmid DNA that is transported by unknown mechanisms to the nucleus. A limitation of DNA liposome complexes has been endosomal degradation; current research on liposome technology has focused on mechanisms to enhance cell entry and reduce degradation in the cytoplasm.
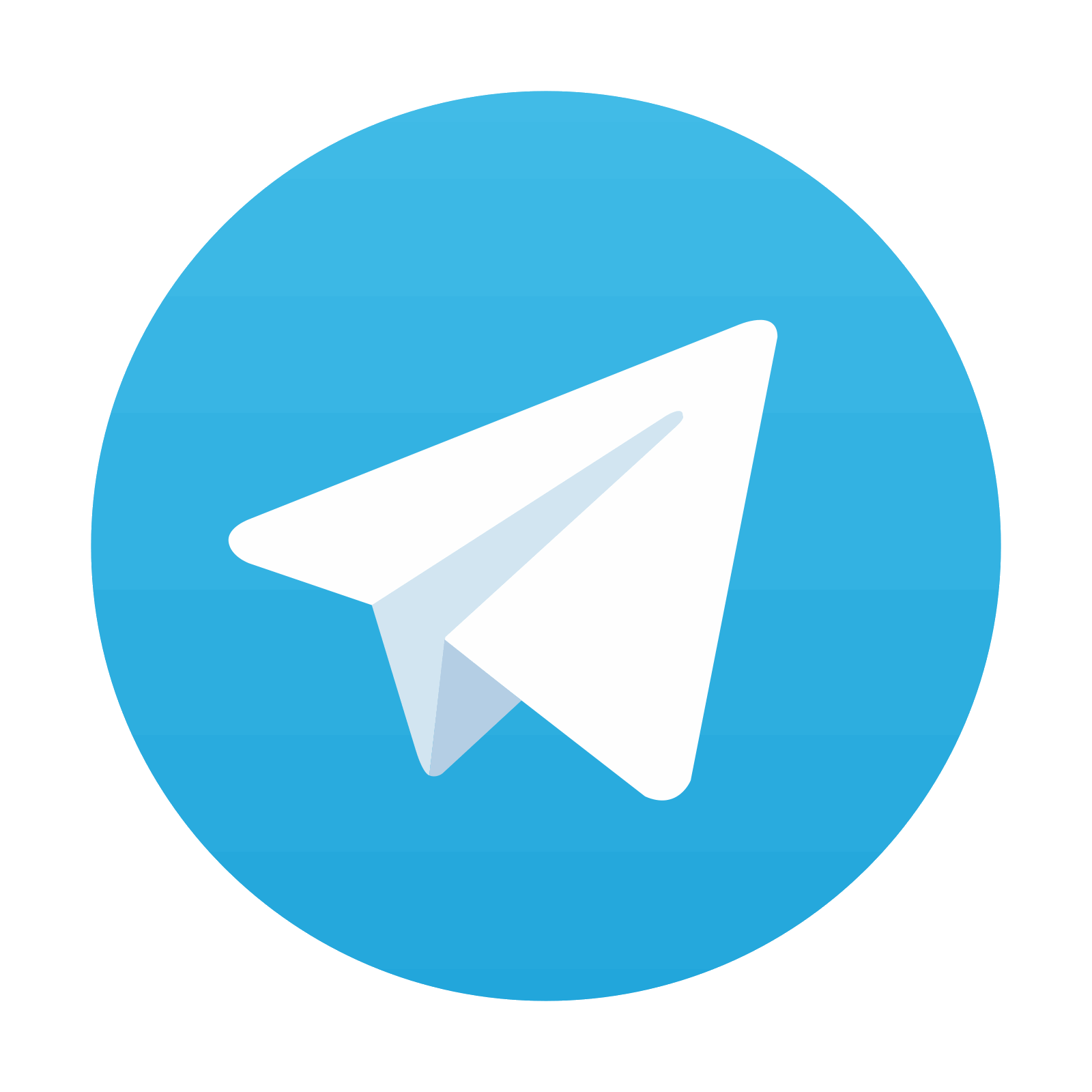
Stay updated, free articles. Join our Telegram channel
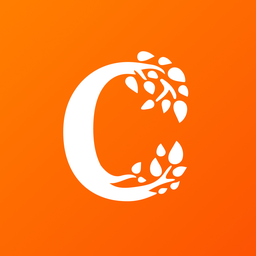
Full access? Get Clinical Tree
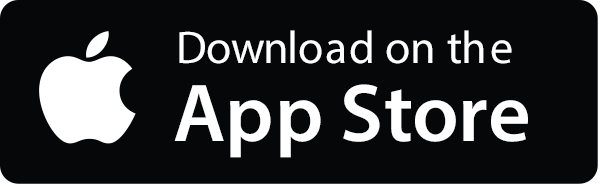
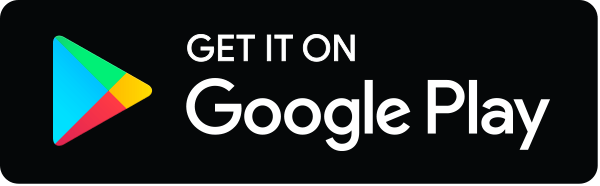
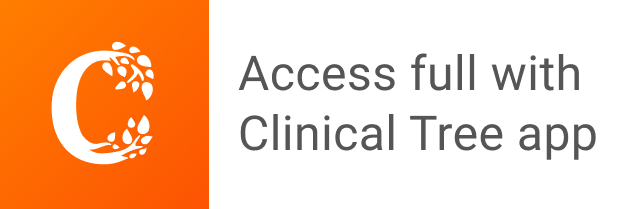