After reading this chapter you will be able to: Gas movement between the lungs and tissues occurs via simple diffusion (see Chapters 6 and 8). Figure 11-1 shows the normal diffusion gradients for O2 and CO2. For O2, there is a stepwise downward “cascade” of partial pressures from the normal atmospheric inspired partial pressure of O2 (PiO2) of 159 mm Hg to a low point of 40 mm Hg or less in the capillaries. The intracellular PO2 (approximately 5 mm Hg) provides the final gradient for O2 diffusion into the cell. PACO2 = Alveolar CO2 tension (mm Hg) Likewise, PACO2 decreases if CO2 production decreases or alveolar ventilation increases. Normally, complex respiratory control mechanisms maintain PACO2 within a range of 35 to 45 mm Hg under various conditions (see Chapter 14). If CO2 production increases, as with exercise or fever, ventilation automatically increases to maintain PACO2 within normal range. FiO2 = Fraction of inspired O2 (decimal) PB = Barometric pressure (mm Hg) 47 = Water vapor tension (in mm Hg) at 37° C The equation component FiO2 × (PB − 47) is a simple application of Dalton’s law: The accompanying Mini Clini provides an example of how to use the alveolar air equation. Because both water vapor tension and PAN2 remain constant, the only partial pressures that change in the alveolus are O2 and CO2. Based on the alveolar air equation, if FiO2 remains constant, PAO2 must vary inversely with PACO2.2–4 PACO2 itself varies inversely with the level of alveolar ventilation. For a constant CO2 production, a decrease in As described in Chapter 6, diffusion is the process whereby gas molecules move from an area of high partial pressure to an area of low partial pressure. To diffuse into and out of the lung and tissues, O2 and CO2 must move through significant barriers. The barrier to gaseous diffusion in the lung is the alveolar-capillary membrane. For CO2 or O2 to move between the alveoli and the pulmonary capillary blood, the following three barriers must be penetrated: (1) alveolar epithelium, (2) interstitial space, and (3) capillary endothelium. In addition, to pass into and out of the red blood cells (RBCs), these gases also must traverse the erythrocyte membrane.5,6 For gas exchange to occur between the alveoli and pulmonary capillaries, a difference in partial pressures (P1 − P2) must exist. Figure 11-3 shows the size and direction of these gradients for O2 and CO2. In the normal lung, the alveolar PO2 averages approximately 100 mm Hg, whereas the mean PCO2 is approximately 40 mm Hg. Venous blood returning to the lungs has a lower PO2 (40 mm Hg) than alveolar gas. The pressure gradient for O2 diffusion into the blood is approximately 60 mm Hg (100 mm Hg − 40 mm Hg). As blood flows past the alveolus, it takes up O2 and moves to the left atrium with a PO2 close to 100 mm Hg in healthy people. For blood leaving the pulmonary capillary to be adequately oxygenated, it must spend sufficient time in contact with the alveolus to allow equilibration.5,8 If the time available for diffusion is inadequate, blood leaving the lungs may not be fully oxygenated. The diffusion time in the lung depends on the rate of pulmonary blood flow. As depicted in Figure 11-4, blood normally takes approximately 0.75 second to pass through the pulmonary capillary. This time is more than enough to ensure complete diffusion of O2 across the alveolar-capillary membrane normally. In clinical practice, knowledge of DL can be helpful in evaluating certain diseases. DL is the bulk flow of gas (ml/min) that diffuses into the blood for each 1-mm Hg difference in the pressure gradient. Although O2 can be used to measure DL, low concentrations (0.1% to 0.3%) of carbon monoxide are used more commonly. Chapter 19 provides details on the technique for measuring DL and its diagnostic use. A shunt is the portion of the cardiac output that returns to the left heart without being oxygenated by exposure to ventilated alveoli. Two right-to-left anatomic shunts exist in normal humans: (1) bronchial venous drainage and (2) thebesian venous drainage (see Chapters 8 and 9). A right-to-left shunt causes poorly oxygenated venous blood to move directly into the arterial circulation (venous admixture), reducing the O2 content of arterial blood. Together, these normal shunts account for approximately three-fourths of the normal difference between PAO2 and PaO2. The remaining difference is a result of normal inequalities in pulmonary ventilation and perfusion.5 The normal respiratory exchange ratio of 0.8 assumes that ventilation and perfusion in the lung are in balance, with every liter of alveolar ventilation ( Figure 11-5 shows graphs of the effect of As the
Gas Exchange and Transport
Describe how oxygen and carbon dioxide move between the atmosphere and tissues.
Identify what determines alveolar oxygen and carbon dioxide pressures.
Calculate the alveolar partial pressure of oxygen at any given barometric pressure and fraction of inspired oxygen.
State the effects that normal regional variations in ventilation and perfusion have on gas exchange.
Describe how to compute total oxygen content for arterial blood.
State the factors that cause the arteriovenous oxygen content difference to change.
Identify the factors that affect oxygen loading and unloading from hemoglobin.
Describe how carbon dioxide is carried in the blood.
Describe how oxygen and carbon dioxide transport are interrelated.
Describe the factors that impair oxygen delivery to the tissues and how to distinguish among them.
Diffusion
Whole-Body Diffusion Gradients
Determinants of Alveolar Gas Tensions
Alveolar Carbon Dioxide
= Rate of CO2 produced (in ml/min standard temperature and pressure, dry [STPD])
= Alveolar ventilation (ml/min body temperature and pressure, saturated [BTPS])
Alveolar Oxygen Tensions
Changes in Alveolar Gas Partial Tensions
simultaneously increases PACO2 and decreases PAO2, whereas an increase in
has the opposite effect (Figure 11-2). However, ventilation can be increased only so much. Neural control mechanisms and the increased work of breathing prevent decreases in PACO2 much below 15 to 20 mm Hg. Whenever a patient is breathing room air at sea level, the RT should not expect to see a PaO2 greater than 120 mm Hg during hyperventilation. PaO2 values greater than 120 mm Hg indicate that the patient is breathing supplemental O2. The accompanying Mini Clini presents a clinical application of these principles.
Mechanism of Diffusion
Barriers to Diffusion
Pulmonary Diffusion Gradients
Time Limits to Diffusion
Normal Variations from Ideal Gas Exchange
Anatomic Shunts
Regional Inequalities in Ventilation and Perfusion
) matched by approximately 1 L of pulmonary capillary blood flow (
). Any variation from this perfect balance alters gas tensions in the affected alveoli. As previously discussed, changes in
affect PACO2, which alters PAO2. Changes in blood flow also alter alveolar gas pressures. If blood flow to an area of the lung increases, CO2 coming from the tissues is delivered faster, causing an increase in PACO2 if minute ventilation remains the same. At the same time, O2 is taken up by the capillaries faster than restored by ventilation, causing a decrease in alveolar PAO2. Decrease in pulmonary capillary blood flow has the opposite effect (i.e., decrease in PACO2 and increase in PAO2) assuming minute ventilation remains the same.5,7,8
Effect of Alterations in Ventilation/Perfusion Ratio
changes on the respiratory exchange ratio (R), plotting all possible values of PAO2 and PACO2. When ventilation and perfusion are in perfect balance (
= 0.99), R equals 0.8. At this point, PAO2 and PACO2 values equal the ideal values of 100 mm Hg and 40 mm Hg.
increases above 1.0 (following the curve to the right), R increases. The result is a higher PAO2 and lower PACO2. At the extreme right of the graph, perfusion is zero (
= ∞). Areas with ventilation but no blood flow represent alveolar dead space (see Chapter 10). The makeup of gases in these areas is similar to that of inspired air (PO2 = 150 mm Hg; PCO2 = 0 mm Hg).
Gas Exchange and Transport
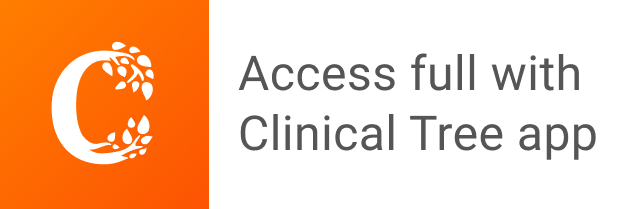