Abstract
This chapter discusses the fundamentals of intracardiac mapping as it relates to the mapping and ablation of cardiac arrhythmias. The initial sections are dedicated to the basis and methodology of intracardiac, extracellular recording techniques; this is followed by a description of intracardiac electrograms as recorded in the normal myocardium, and subsequently by a description of intracardiac signals in the abnormal heart. The remainder of the chapter is dedicated to the application of various endocardial mapping techniques, including activation, pace, and entrainment mapping, in the diagnosis and ablation of atrial and ventricular arrhythmias in both normal and diseased hearts.
Keywords
bipolar signals, entrainment mapping, intracardiac mapping, pacing maneuvers, recording techniques, unipolar signals
Key Points
- •
Intracardiac electrograms provide timing and morphologic information.
- •
Local tissue activation is best identified by the point of maximal downslope of unipolar electrograms and maximal amplitude of bipolar electrograms.
- •
Cardiac mapping techniques include activation mapping, pace mapping, entrainment mapping, and computerized mapping (sometimes called substrate mapping).
- •
Atrial and ventricular pacing maneuvers performed in sinus rhythm and during tachycardia can be used to differentiate and diagnose cardiac arrhythmias.
This chapter discusses the fundamentals of intracardiac mapping as it relates to the mapping and ablation of cardiac arrhythmias. The initial sections are dedicated to the basis and methodology of intracardiac, extracellular recording techniques; this is followed by a description of intracardiac electrograms as recorded in the normal myocardium, and subsequently by a description of intracardiac signals in the abnormal heart. The remainder of the chapter is dedicated to the application of various endocardial mapping techniques, including activation, pace, and entrainment mapping, in the diagnosis and ablation of atrial and ventricular arrhythmias in both normal and diseased hearts. Computerized mapping is discussed in Chapter 7 .
Underlying Basis for the Extracellular Electrogram
Cardiac electrical activity originates from activation of ion channels across cell membranes. The cardiac action potential is generated within individual cells and reflects cardiac electrical activation. Although some net charge flow occurs in the extracellular space, most cardiac electrical activity is generated within individual myocardial cells. Extracellular electrodes record potentials generated in the extracellular space and therefore differ markedly from action potentials recorded intracellularly. The differences are caused not only by differences in recording location (extracellular vs. intracellular) but also by the inherent summation of electrical activity from multiple cells that occurs when an extracellular potential is generated.
The field of view of extracellularly recorded electrograms reflects the relative contribution of individual cells both near to and far from recording electrodes that generate extracellular potentials. Computer modeling studies have created simulated extracellular potentials using various assumptions regarding intracellular action potentials. Factors that affect the field of view of recording electrodes are whether the recordings are unipolar or bipolar, interelectrode distance (for bipolar recordings), electrode size and composition, and inherent myocardial properties such as tissue resistivity and space constant. In view of the summation of intracellular potentials that occurs to generate extracellular potentials, some fundamental questions regarding cardiac mapping require further investigation. For example, when one asks the activation time determined from extracellular potentials, there may not be a single answer, because different cells within the field of view of an extracellular recording electrode may be activated at different times. Thus uniform rules regarding interpretation of extracellular electrograms need to account for differences in underlying physiology, and different rules may be appropriate in different circumstances. For example, it has generally been accepted that the HV interval should be measured from the onset of the His bundle electrogram. The basis for this approach is that regardless of the location of the recording electrode, one wishes to determine the onset of activation within the His bundle to best evaluate conduction time within the His–Purkinje system. By contrast, mapping of tachycardia origin uses techniques such as the baseline crossing in a bipolar electrogram that seeks to determine not the onset of activation but the occurrence of activation at a specific location, and in most cases to predict the effects of ablation at that site. Thus understanding the physiology that generates extracellularly recorded potentials and the purpose of a particular mapping technique is required to determine the best theoretical as well as practical techniques to use for intracardiac mapping.
Electrogram Recording: Amplification, Filtering, and Digitization of Signals
Physiologic signals acquired through intracardiac electrodes are typically less than 10 mV in amplitude and therefore require considerable amplification before they can be digitized, displayed, and stored. In most modern electrophysiology laboratories, the signal processor (filters and amplifiers), visualization screen, and recording apparatus are often incorporated as a computerized laboratory recording system. The amplifiers used for recording intracardiac electrograms must have the ability to gain modification as well as to alter both high-band–pass and low-band–pass filters to permit appropriate attenuation of the incoming signals.
After amplification, signals are digitized and filtered by a computerized data-acquisition system and are written to a hard disk or optical drive, while displaying signals in real time on a monitor. Digitization is a form of data reduction, whereby an analog waveform is sampled at a constant rate (sampling rate) by an analog-to-digital (A/D) converter. The amplitude of the analog waveform is translated into a binary number (e.g., 8-, 10-, or 12-bit conversion resolution of the A/D converter), which represents the full dynamic input range of the A/D converter (typically ±2.5 or ±10 V). Sampling rates of 600 Hz or more allow for the recording of most of the data contained in the intracardiac electrogram waveforms, with most modern mapping systems sampling at or about 1000 Hz. The ideal system should be able to provide a variety of display configurations with a wide range of sweep speeds (most modern systems can display up to 400 mm/second) and should allow adjustment of the size and gain and other characteristics of the amplified electrogram.
Filtering is an important aspect of electrogram processing ( Table 6.1 ). High-pass filters eliminate components below a given frequency (allowing signal content of higher frequency to pass without attenuation). In the surface electrocardiogram (ECG), components such as the T wave are of relatively low frequency, and high-pass filtering of 0.05 to 0.1 Hz is used to preserve these components while eliminating baseline drift. When examining bipolar intracardiac electrograms, high-frequency components are of the most interest, and high-pass filtering of 30 to 50 Hz is used to eliminate the low-frequency components. Unipolar electrograms usually go through a high-pass filter of 0.05 Hz, however, because the polarity of the signal (which reflects the direction of myocardial activation) and the signal morphology (and therefore low-frequency components) must be preserved. To eliminate noise at higher frequencies, low-pass filters are generally set to about 500 Hz for intracardiac signals (because there are essentially no intracardiac signals of interest much above 300 Hz). Notch filters remove specific frequencies such as 60-Hz noise from power supplies. Fig. 6.1 shows the effect of different filter settings on intracardiac atrial, ventricular, and His–Purkinje signals.
Recording | High Pass | Low Pass |
---|---|---|
Surface electrocardiogram | 0.05–0.1 Hz | 100 Hz |
Bipolar intracardiac | 30–50 Hz | 300–500 Hz |
Unipolar intracardiac | DC, 0.05 Hz | >500 Hz |

Unipolar and Bipolar Signals
The morphology and amplitude of the recorded electrograms depend on (1) the type of normal or abnormal depolarization responsible for the electrical potential and on local myocardial characteristics such as ischemia or infarction; (2) the orientation of the activation wave front in relation to myocardial fiber orientation ; (3) the distance between the source of the potential and the recording electrode; (4) the size, configuration, and interpolar distance of the recording electrode ; (5) the orientation of the wave front in relation to the poles of a bipolar electrode; (6) the conducting medium in which electrograms are recorded ; and (7) other factors.
The unipolar electrogram is recorded as the potential difference between a single electrode in direct contact with the heart (exploring electrode) and an indifferent electrode, which is placed at a distance from the heart (such as in the inferior vena cava) or at the Wilson central terminal. The recording is therefore not truly unipolar because all recordings depend on voltage differences between two poles; the unipolar designation signifies that one of the poles is distant from the heart. During cardiac activation, the approach of the dipole of an activation wave front toward an exploring electrode gives a small positive deflection, and its passage gives a rapid deflection in the negative direction, with a final return to baseline. The amplitude of the unipolar electrogram is proportional to the area of the dipole layer and the reciprocal value of the square of the distance between the dipole layer and the recording site. Thus the unipolar electrogram records a combination of local and distant electrical events, with the contribution of distant electrical events decreasing in proportion to the square of the distance from the exploring electrode. As mentioned earlier, extracellular recordings are not synonymous with intracellular microelectrode recordings. Nonetheless, in normal myocardium with relatively homogeneous conduction and repolarization, several studies (as well as theoretical models) have shown conformity of activation times between intracellular microelectrode and extracellular recordings ( Fig. 6.2 ), with the maximal downslope of the unipolar electrogram coinciding with the upstroke of the transmembrane potential. Therefore at least in normal hearts, there is agreement on using the maximal downslope of the unipolar electrogram for activation detection; there is significant controversy about the optimal value of the slope threshold, however, with recommended thresholds from different studies ranging from − 0.2 to − 2.5 mV per ms; the large range can be at least partially attributed to the fact that these studies were performed under a variety of different baseline conditions in normal, acutely ischemic as well as chronically infarcted hearts (animal and human).

The bipolar electrogram is recorded as the potential difference between two closely spaced electrodes in direct contact with the heart; it can be calculated as the difference between two unipolar electrograms at each of the two electrode sites ( Fig. 6.3 ). In the electrophysiology laboratory, this is typically done with analog amplifiers rather than with digital subtraction between unipolar signals. The amplitude of the bipolar electrogram is inversely proportional to the third power of the distance between recording site and dipole.

The major advantage of bipolar recordings lies in the distinction between local and distant activity. A limitation of bipolar electrograms is their directional sensitivity; as a result, if the activation wave front is parallel in relation to the electrode pair, the bipolar spike will be of maximal amplitude, whereas if it is perpendicular, both electrodes will record the same waveform at the same time, and no spike will result. In addition, activation at the two poles of the bipolar electrogram is not simultaneous, making activation detection more difficult in bipolar electrograms. The following criteria have been suggested for activation detection in bipolar electrograms: (1) the maximal absolute value of the bipolar electrogram; (2) the first elevation of the electrogram of more than 45 degrees from the baseline (obviously subject to display gain); (3) the baseline crossing with the steepest slope; and (4) morphologic algorithms that search for symmetry in the bipolar waveform. Of these, the maximal amplitude of the bipolar electrogram is the most easily measured and has been shown to coincide closely with the maximal downslope of the unipolar electrogram (and the maximal upstroke of the monophasic action potential).
Recording Artifacts
The identification of artifactual electrograms is of crucial importance in any system of cardiac mapping and may have major influence on the final interpretation of an activation sequence, such as whether a fractionated electrogram represents a motion artifact or local activation in an assumed zone of slow conduction ( Table 6.2 ). Typical recording artifacts induced at the myocardium–electrode interface are (1) polarization of electrodes, which can cause slow shifts of the baseline of the signals; (2) local myocardial injury resulting from inappropriate pressure by recording electrodes ; (3) motion artifacts, which are often rhythmic and linked to cardiac events, therefore simulating fractionated electrograms, or which can be sudden shifts of potential that may be misinterpreted as activations by computer algorithms; (4) poor contact between electrode and myocardium, leading to heavier weighing of far-field effects and increased 50- or 60- Hz noise; (5) potentials produced by two electrodes from different catheters touching each other; and (6) repolarization signals masquerading as a mid-diastolic potential. Finally, intermittent recording of actual signals that do no repeat with every cycle (most typically signals that appear on alternate cycles during tachycardia) are problematic for computerized mapping algorithms. Ensuring good contact between the recording electrode and the underlying myocardium (to remove far-field effects), eliminating all possible sources of noise, including adequate grounding for 50 to 60 Hz (and if necessary the use of notch filters), and locating preamplifiers and amplifiers as close as possible to the mapped heart may all help eliminate most of the artifacts that are seen in the clinical electrophysiology laboratory. Undue pressure by the recording catheter on the underlying myocardium can be reflected by the appearance of ST-segment elevation on the unipolar electrogram; slight catheter repositioning usually results in resolution of the ST segment to baseline. Motion artifact (from the patient or the surrounding environment) can be minimized by preventing contact of perfusion pumps and other equipment capable of generating cyclical noise with the patient table and mapping equipment.
Cause | Manifestation |
---|---|
Electrode polarization | Electrogram drift |
Excessive contact pressure | ST elevation |
Catheter motion | Fractionation |
Poor contact | Low amplitude |
Contact with other catheters | High-frequency signals |
Repolarization | Late or mid-diastolic potentials |
Electromagnetic interference | High-frequency noise |
Poor grounding | High-frequency noise |
Characteristics of Intracardiac Signals: Normal Heart
Unipolar endocardial electrograms obtained from experiments in normal canine hearts and from the human atrium and ventricle are characterized by a QS morphology with a rapid downstroke in the first part of the QRS complex (intrinsic deflection). Bipolar endocardial electrograms in the normal human left ventricle from catheters with 10-mm interelectrode distance have amplitudes greater than 3 mV and durations less than 70 ms, and no split, fractionated electrograms are found. Unipolar and bipolar electrograms in diseased myocardium are typically characterized by slower upstrokes and fragmentation (see later discussion).
Although slow conduction and fractionated, low amplitude signals are not as widespread in the normal heart compared with the diseased atrium or ventricle, low-amplitude, high-frequency electrograms have been well described at the thoracic vein–atrium junction, within both the pulmonary veins and the vena cava, as well as at other sites in the normal heart, such as the crista terminalis and coronary sinus.
Electrical Abnormalities in the Absence of Structural Heart Disease
Electrograms need not be entirely normal even in the absence of overt fibrosis or fatty replacement. For example, low-amplitude, fractionated bipolar electrograms may be seen at the earliest activation sites of focal tachycardias, even in the presence of a normal looking unipolar electrogram. Fig. 6.4 shows the bipolar electrogram at the successful ablation site of two different focal tachycardias in the absence of any overt structural heart disease: a focal tachycardia originating from the coronary sinus ostium and a focal ventricular tachycardia (VT) arising from the inferolateral left ventricle. Late potentials during sinus rhythm are also noted in hearts that are otherwise morphologically normal; Fig. 6.5 shows late potentials in the right ventricle of a patient who had exercise-induced left bundle branch block (LBBB) superior axis VT, but with no evidence of scar or any other abnormality on ECG or cardiac magnetic resonance imaging (MRI).


The basis for these potentials is not entirely clear. In the absence of overt scar on sensitive imaging modalities such as MRI, it is tempting to speculate that at least some of the delay seen in these electrograms may be functional in nature. In fact, functional anisotropic conduction is well described in the normal heart (e.g., at the crista terminalis) and may be responsible for the creation of macroreentrant circuits, such as isthmus-dependent atrial flutter. Anisotropic conduction has also been demonstrated within the pulmonary veins and the superior vena cava. Fig. 6.6 shows that the conduction times into and out of the pulmonary vein can be very different, which is an example of anisotropic conduction.

Conceivably, therefore at least some of the focal tachycardias described in the normal heart may be microreentrant in origin. Other factors that have been postulated are poor intercellular coupling, such as that caused by decreased gap junctions at sites of origin of focal tachycardias, leading to decreased electrotonic inhibition of the focus by surrounding tissue. In general, electrograms at successful ablation sites for focal tachycardias are less than 50 ms presystolic. However, pulmonary vein tachycardias are usually more than 50 seconds presystolic, at least partially on account of the slow conduction noted in this area.
Fractionated, bipolar electrograms (both during tachycardia and during sinus rhythm) have also been well described in idiopathic VT arising from the left ventricular septum. In fact, it is the presence of these Purkinje-like potentials some distance away from the septum that has led some to postulate a reentrant circuit of considerable size as the basis for this VT. Split electrograms may sometimes be recorded in the normal heart, such as in the posteroseptal right atrium from conduction block across the Eustachian ridge.
Electrogram Abnormalities in the Presence of Structural Heart Disease
The signature of scar in the atrium or ventricle is a low-amplitude, high-frequency electrogram (during sinus rhythm or during tachycardia), with the duration and amplitude of the electrogram showing some correlation with the degree of slow conduction in and around the area of fibrosis. Low-amplitude, fractionated electrograms may be recorded during or after the QRS complex and are typically less than 1 mV in amplitude; these can be recorded during sinus rhythm and VT. The characteristic unipolar electrogram in the setting of chronic myocardial scar, such as myocardial infarction, may show a single, rapid biphasic deflection of RS morphology following a wide QS potential, a double RS deflection, or fragmentation with multiple deflections. Continuous, low-amplitude electrical activity, such as a fractionated electrogram spanning the entire tachycardia cycle length (TCL), can also be seen in the setting of myocardial scar or disease. Fig. 6.7 shows an example of low-amplitude continuous activity at a successful ablation site for a reentrant atrial tachycardia and flutter in a patient who had undergone Mustard repair for D-transposition of the great arteries.

Animal studies performed in the setting of experimental chronic myocardial infarction have examined the intracellular basis of the previously mentioned changes on the extracellularly recorded electrograms and have demonstrated that action potential amplitude and upstroke are similar to those of normal myocardium. Despite the presence of normal action potentials, slow and discontinuous conduction is present, which may provide an electrophysiologic and anatomic basis for arrhythmias. One explanation of the low-amplitude, prolonged duration, and notched extracellular potentials in the infarcted areas is that the slow conduction represents activation of normal cells separated by areas of fibrous tissue producing discontinuities in conduction. Kadish and colleagues used vector mapping, a technique based on summing orthogonally bipolar electrograms, to determine the direction of activation in cardiac tissue and showed that although vector loops recorded from normal myocardial tissue were found to be smooth and pointing in a single direction, those recorded from areas of healed myocardial infarction were notched, irregular, and occasionally pointing in more than one direction. Using the vector technique, the authors demonstrated complex activation patterns in areas where abnormal extracellular electrograms are recorded in which discrete activation times may be difficult to identify, such as in areas of fractionated electrograms where multiple peaks are present.
Ischemic heart disease is by far the most common cause of scar in the ventricle, although less common conditions such as arrhythmogenic right ventricular (RV) dysplasia and cardiomyopathy may demonstrate similar electrophysiologic characteristics because of fibrofatty replacement in the right (and occasionally left) ventricle. Fibrosis has also been described in dilated cardiomyopathy but is seen far less common than in ischemic heart disease. In the atrium, scar is commonly the result of surgery for congenital heart disease, and atrial septal defect repair, tricuspid atresia (Fontan repair), tetralogy of Fallot repair, and transposition of great arteries (Mustard–Senning repair) are the most common reasons for scar in the right atrium. Left atrial surgery can create substrate for left or right atrial reentry, the latter likely on account of cannulation sites during bypass. Similarly, extensive catheter ablation with multiple linear or multiple areas of focal lesions may also create substrate for subsequent development of left or right atrial tachyarrhythmias. Please see Chapter 12 for details.
As mentioned earlier, the low-amplitude electrograms during sinus rhythm are often noted late in systole; Fig. 6.8 shows an example of late systolic potentials in the right ventricle of a patient with arrhythmogenic RV dysplasia. Such low-amplitude, high-frequency electrograms are thought to underlie the late potentials that are seen on a positive signal-average ECG. Lately, there has been a renewed interest in local abnormal ventricular activities (LAVAs), some occurring before the end of QRS, in patients with structural heart disease. Jaïs and colleagues showed that a procedural end point of LAVA elimination was associated with mortality benefit and freedom from VT, compared with standard methods of VT mapping and ablation. However, even though late potentials are typically seen in and around an area of scar or infarction and are frequently noted at or close to sites of successful ablation (of reentrant tachycardias), late potentials have not always been shown to be a clear predictor of ablation success. They may lack specificity in that they may also be found at sites that are remote from a successful ablation site (indicating diseased myocardium in other areas). The presence of late potentials can also be dependent on the activation timing of the anatomic substrate with LAVA seen more commonly in the epicardium rather than the endocardium and beyond the septal area. The ability to distinguish late potentials (sometimes needing to decouple LAVA from the basic ventricular electrogram) is also dependent on conduction velocity in the diseased myocardium. A list of abnormal electrogram morphologies and the possible interpretations is given in Table 6.3 .

Morphology | Definition | Interpretations |
---|---|---|
Low amplitude | <0.5 mV in the atrium and <1.5 mV in the ventricle | Myocardial infarction, fibrosis, infiltrative process, poor contact, far-field |
Fractionated | Prolonged (>70 ms), low-amplitude potential with multiple peaks or multiple baseline crossings | Peri-infarction, slow conduction, catheter motion, arborized myocardial connection |
Split | >70 ms duration with two components separated by isoelectric interval | Local conduction block, surgical scar, slow conduction |
Late component | Potential occurs after end of surface QRS or P wave | Delayed activation, line of block, slow conduction |
Continuous | Absence of isoelectric interval throughout diastole | Slow conduction, electromagnetic interference artifact |
Mid-diastolic | Potential occurs in mid-diastole bounded by isoelectric intervals | Protected isthmus, bystander connection, repolarization, or motion artifact |
Low frequency | Low depolarization velocity ( dV / dt ) | Far-field, artifact |
Monophasic action potential | Injury current pattern | Excessive contact pressure, local tissue injury (unipolar) |
Endocardial Mapping Techniques
Contact endocardial mapping can be performed during sinus rhythm as well as during tachycardia. Mapping techniques can be broadly categorized as follows: (1) activation sequence mapping; (2) fractionated local electrogram and voltage mapping; (3) pace mapping; (4) entrainment mapping; and (5) miscellaneous pacing maneuvers (performed during sinus rhythm or tachycardia). Although these techniques are usually used in combination, the relative utility of each depends on arrhythmia inducibility, hemodynamic and cycle length stability of the induced arrhythmia, underlying substrate (i.e., normal vs. diseased heart), and arrhythmia mechanisms, among other factors. For example, entrainment can be used only in the setting of a sustained, rather regular reentrant arrhythmia. The previous two sections have described the electrogram and voltage characteristics in normal and diseased hearts. The following paragraphs describe the underlying basis for the remainder of the techniques and highlight their use (singly or in combination) in the diagnosis and ablation of atrial and ventricular arrhythmias.
Activation Sequence Mapping
The most commonly used method of identifying the optimal ablation site for a tachyarrhythmia is activation mapping to locate the site of earliest endocardial activation or to determine the activation sequence during the tachycardia. Focal arrhythmias are typically characterized by presystolic timings pre-P or pre-QRS less than 50 ms. By contrast, reentrant arrhythmias, both in the normal and in diseased hearts, typically demonstrate diastolic activity that is significantly earlier than 50 ms. In fact, for reentrant arrhythmias, intracardiac activity should be present at some point in the heart throughout the cardiac cycle. Electrograms are typically recorded through at least 70% of the TCL in macroreentrant arrhythmias. In the normal heart, such as in the setting of subeustachian isthmus–dependent typical atrial flutter, the macroreentrant circuit is of considerable size—bound by the two anatomic barriers of the crista terminalis and the tricuspid annulus—and need not demonstrate the low-amplitude, high-frequency electrograms that are typical of scar-related reentrant arrhythmias. In a reentrant VT in the setting of myocardial scar, a zone of slow conduction over a pathway of possibly quite complex geometry (e.g., nonlinear, nonhomogeneously anisotropic) provides the diastolic limb of the reentrant circuit. After emerging from the zone of slow conduction, the wave front propagates rapidly throughout the ventricles to generate the QRS complex. Thus early or presystolic activation occurs in close proximity to the exit region from the zone of slow conduction.
What is considered to be early activation and a suitable ablation site in a focal arrhythmia (30–50 ms presystolic) may not necessarily be early activation in a reentrant arrhythmia. Reentrant atrial tachycardias or VTs are typically characterized by earlier electrogram timing in diastole, with mid-diastolic (or even earlier) potentials (discussed later) having been correlated with successful ablation sites for these arrhythmias. Fig. 6.9 shows an example of atrial tachycardia that was induced in a patient with no known atrial disease; mapping with the ablation catheter revealed electrograms in and around the tricuspid annulus that were significantly more than 50 ms presystolic, thereby raising suspicion for a macroreentrant tachycardia; a halo duodecapolar catheter was subsequently inserted into the right atrium and showed an activation sequence consistent with counterclockwise reentry around the tricuspid annulus (see Fig. 6.9B ). Entrainment maneuvers confirmed the diagnosis and led to successful ablation of the arrhythmia at the cavotricuspid isthmus (CTI).

Mid-Diastolic Potentials
If an intervening isoelectric segment exists between QRS complexes during VT, the prepotentials are called mid-diastolic potentials ( Fig. 6.10 ); it has been suggested that they identify the region of slow conduction. These prepotentials may precede the major ventricular deflection by 10s to 100s of milliseconds.

It is important to demonstrate that during initiation and resetting of an arrhythmia, loss of the mid-diastolic potential is associated with termination of the arrhythmia. Although there are some recent data to support the specificity of diastolic potentials during sinus rhythm as the potentially successful ablation sites (i.e., critical isthmus sites during VT), most studies have looked at diastolic potentials during sustained tachycardia as markers of a protected isthmus.
Mid-diastolic potentials that do not dynamically precede the QRS complex of the VT may represent blind-end alleys of late activation or motion artifact. In one study, almost continuous electrical activity spanning the diastolic segment was frequently seen in patients with VT because of ischemic heart disease or arrhythmogenic RV dysplasia. However, in 85% of patients, the episodes of continuous electrical activity could be dissociated from the VT. Such behavior is presumably caused by slow and stuttering conduction into areas of diseased myocardium not essential for ongoing tachycardia.
Unipolar Potential Mapping
Unipolar electrograms provide useful adjunctive mapping information to bipolar electrograms during mapping for focal atrial and VTs. Amerandral and associates noted that the presence of a QS complex was highly sensitive in identifying successful ablation sites. However, nearly 70% of unsuccessful sites also manifested a QS unipolar complex, with QS complexes being recorded more than 1 cm away in 65% of patients. The unipolar electrogram morphology with standard ablation electrodes is therefore a highly sensitive but nonspecific marker for target sites in patients with idiopathic RV and other VTs as well as atrial tachycardias. Unipolar electrograms are of limited utility, however, in the setting of myocardial scar or fibrosis, because the criteria established for unipolar activation (i.e., peak depolarization velocity (dV/dt)) cannot be relied on in the presence of slow, disordered conduction, with the onset of unipolar recordings in this setting frequently showing a delayed initial deflection.
Much has been written about the utility of the unipolar electrogram in localizing insertion and successful ablation sites for accessory pathways. Note that most of these data are for anterograde mapping in the setting of overt preexcitation, although some investigators have demonstrated the utility of unipolar mapping for retrogradely conducting accessory pathways. Electrograms characteristic of the insertion sites of accessory pathways have been described in detail in Chapter 23 , Chapter 24 , Chapter 25 , Chapter 26 , Chapter 27 of this book.
Pace Mapping
Pace mapping involves manipulation of the mapping catheter to the region of origin of the tachycardia. Pacing at this site, using the same cycle length as the tachycardia, should generate P waves or QRS complexes resembling those during tachycardia. The greater the degree of concordance between the P wave or QRS morphology during pacing and tachycardia, the closer the catheter is to the exit site of the tachycardia. In addition, the stimulus to QRS interval of more than 40 to 70 ms is an indication of slow conduction.
Pace mapping has advantages over activation mapping in that induction of arrhythmia is not required; as a result, it allows identification of the site of origin when the induced arrhythmia is poorly tolerated or when sustained tachycardia is not inducible by electrophysiologic techniques but when P wave or QRS morphology from a 12-lead ECG is available. However, others have argued that pace mapping is not as sensitive or precise as activation mapping and may be more time consuming. Pace mapping has generally been more useful as a means of corroborating candidate ablation sites, with a combination of activation and pace mapping used to best identify the optimal target site of ablation. For generalized localization of an arrhythmia exit site, such as with substrate mapping of unstable ventricular arrhythmias, matching 10/12 leads may be sufficient. For precise arrhythmia localization, 12/12 leads should match the clinical tachycardia.
Most investigators report successful ablation at sites with identical or nearly identical matches in all 12 surface leads. Differences in QRS configuration between pacing and spontaneous tachycardia in a single lead may be critical. An example is shown in Fig. 6.11 , in which ablation success was achieved (in the left coronary cusp) only at the site of a 12/12 or perfect pace map. Fig. 6.12 shows an example of premature ventricular complexes (PVCs) arising from the right coronary cusp. Unlike in Fig. 6.11A, in which there is a small R wave in the unipolar electrogram, the distal unipolar electrogram in Fig. 6.12A has a QS complex; pace mapping at this site also resulted in a nearly perfect pace map (see Fig. 6.12B ). Ablation at this site resulted in elimination of all PVCs.


The use of single ECG leads or body-surface mapping may improve the precision of pace mapping. Kadish and colleagues examined the spatial resolution of unipolar pacing with respect to the degree of pace-map matching and found that under optimal conditions—that is, when minor differences in configuration (notching, new small component, change in amplitude of individual component, or overall change in QRS shape) in at least one lead were accounted for—the spatial resolution of an exact pace-map match may be less than 5 mm. Bipolar pacing may introduce additional variability in the ventricular paced ECG, but these changes may be minimized by low-pacing outputs and small interelectrode distances (≤5 mm) or by unipolar pacing. Pace mapping should be performed as close as possible to the cycle length of the spontaneous tachycardia to minimize rate-dependent aberration in paced P-wave or QRS configuration (owing to increasing degrees of incomplete repolarization and fusion with the preceding T wave during shorter cycle lengths).
Pace mapping during sinus rhythm has been shown to be less sensitive in the setting of scarred myocardium, even when pacing is performed close to the tachycardia circuit. The activation wave front may take the path of least resistance and, instead of mimicking the tachycardia wave front (through the protected tachycardia isthmus), may activate normal myocardium first, thereby creating a very different pace map. In addition, the boundaries of a protected isthmus may be functional in nature and therefore difficult to replicate with pacing during sinus rhythm. Recently, several investigators have shown that pace mapping may be performed at the infarct border with good sensitivity and specificity and can provide successful target sites for ablation based on the closeness of the pace map to the tachycardia and the stimulus-to-QRS interval (helping outline the critical isthmus from entrance to exit; Fig. 6.13 ).

Entrainment Mapping
Entrainment is one of the most powerful tools in the electrophysiologist’s arsenal that serves the following purposes: (1) allows confirmation of reentry as the underlying mechanism of a sustained arrhythmia; (2) allows localization of an underlying reentrant circuit (e.g., to the right or left atrium in the setting of a reentrant atrial tachycardia); and (3) allows identification of myocardium that is critical to the reentrant circuit (i.e., critical isthmus). The critical isthmus may be narrow enough to be amenable to ablation, with resulting interruption of the tachycardia. A full discussion of resetting curves, entrainment criteria, and the pitfalls of entrainment is beyond the scope of this chapter; the reader is referred to other authoritative texts for a more in-depth study of the principles of entrainment.
Entrainment is continuous resetting of a reentrant circuit that has an excitable gap by a series of stimuli. The presence of entrainment is established when pacing at a rate slightly faster than the TCL accelerates all QRS complexes or P waves (or intracardiac electrograms) to the pacing rate and termination of pacing is followed by resumption of the same tachycardia with the presence of constant surface or intracardiac fusion during pacing (or progressive fusion during pacing at progressively faster cycle lengths). The four criteria for manifest entrainment are (1) constant fusion during overdrive pacing, except for the last captured beat, which is entrained but not fused; (2) progressive fusion during overdrive pacing at different cycle lengths; (3) localized conduction block to a site for one paced beat associated with interruption of the tachycardia, followed by activation of that site by the next paced beat from a different direction and with a shorter conduction time; and (4) a change in conduction time to and electrogram morphology at an electrode recording site when pacing at two different rates during a tachycardia (i.e., the intracardiac equivalent of criterion 2, because it may be difficult to visualize surface criteria in some cases, such as for atrial tachycardia).
Fig. 6.14 shows an example of manifest entrainment (based on intracardiac electrograms); activation around the tricuspid annulus is shown during overdrive pacing of what appeared to be typical, counterclockwise atrial flutter in a patient with a normal heart. Pacing at progressively faster rates demonstrates progressive fusion—one of the diagnostic criteria for entrainment and therefore reentry—which is best demonstrated by a change in activation (as well as timing) of the bipolar electrograms in the distal halo electrodes. In fact, even when changes in timing are subtle, electrogram morphology may provide important clues to a diagnosis.

After entrainment has been demonstrated (i.e., manifest entrainment), it can be used for mapping. Entrainment mapping is centered on obtaining a match between the entrained surface P wave or QRS complex or intracardiac electrograms and tachycardia morphology (concealed entrainment; discussed in a later section) and on obtaining a postpacing interval (PPI; described later) that is equal to the TCL. Although the demonstration of resetting with fusion (or manifest entrainment) confirms reentry, the presence of concealed entrainment allows the ablationist to home in on a protected isthmus—a common site of ablation success. Concealed entrainment is characterized by a failure to demonstrate fusion (i.e., criteria 1, 2, and 4) during pacing from a protected isthmus within a tachycardia circuit ( Fig. 6.15 ) and is associated with a PPI that is equal to the TCL. The PPI is the time between the last pacing stimulus that entrained the tachycardia and the next recorded electrogram at the pacing site. The PPI should be equal to the TCL (within 20 to 30 m) if the pacing site is within a critical part of the reentrant circuit. If the pacing site is outside of the circuit, the PPI should be equal to the TCL plus the time required for the stimulus to propagate from the pacing site to the tachycardia circuit and back. Problems arise when the electrogram at the pacing site is distorted immediately after pacing. To overcome this shortcoming, alternative methods of estimating the PPI have been developed. The first method is used when the electrogram on the pacing or mapping electrode is obscured in the first cycle after the termination of pacing and is given by the following equation:
ER ( N + 1 DIFF Eg ) = [ S − Eg ( N + 1 ) ] − [ L ( N + 2 ) − Eg ( N + 3 ) ]
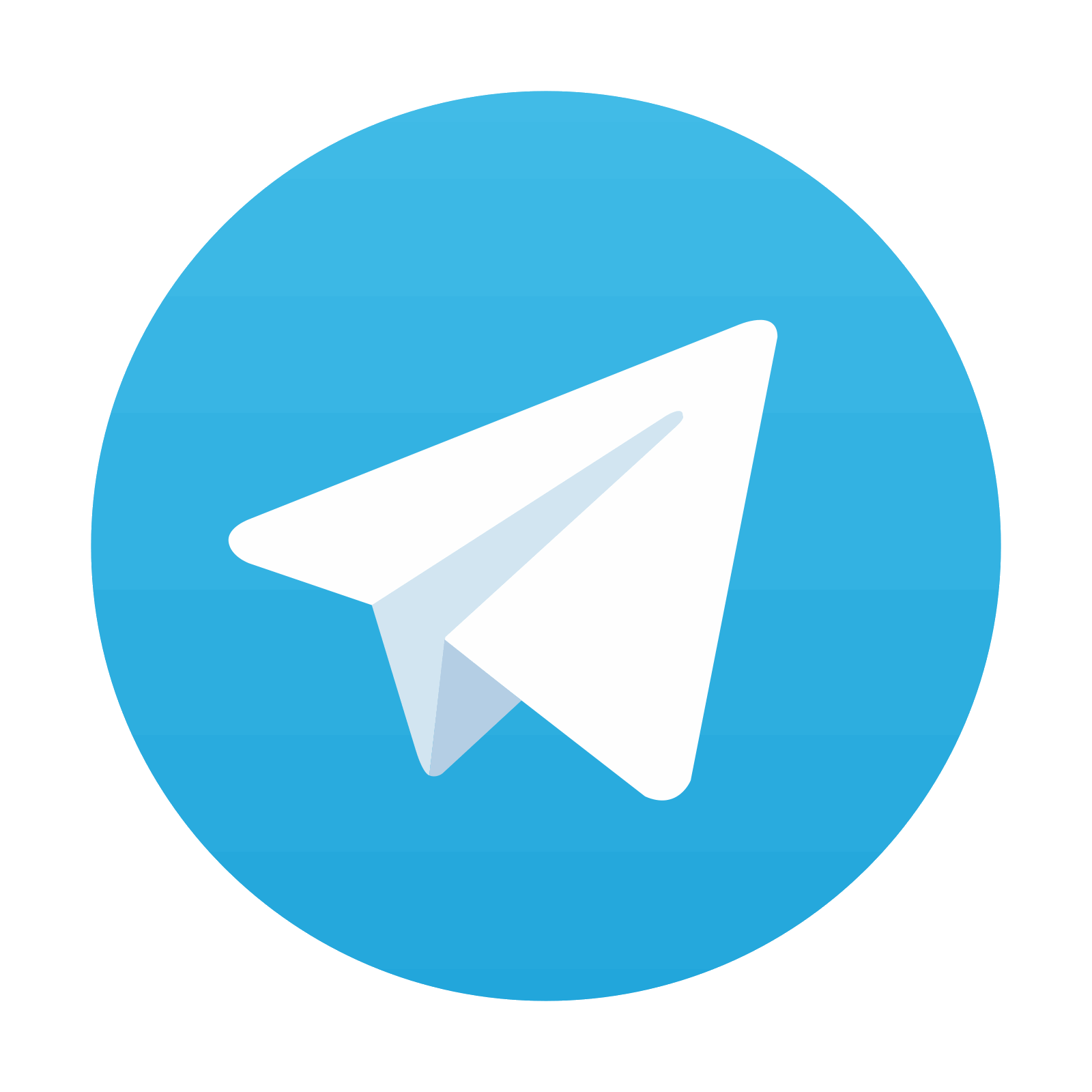
Stay updated, free articles. Join our Telegram channel
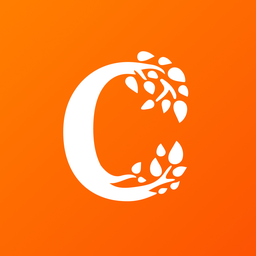
Full access? Get Clinical Tree
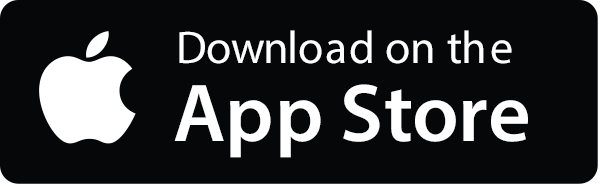
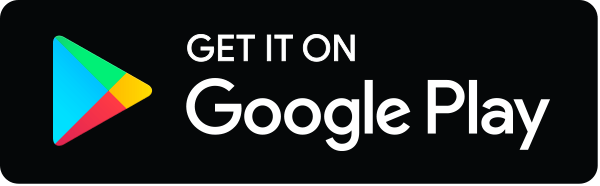