Timothy S. Lancaster, Jacob R. Miller, and Pirooz Eghtesady Washington University School of Medicine, St. Louis Children’s Hospital, St. Louis, MO, USA Our current understanding of fetal cardiovascular physiology derives largely from the pioneering work of a group of South African researchers who relocated to the University of California San Francisco in the mid‐1960s. Notable among them were Dr. Abraham Rudolph and Dr. Michael Heymann, who wrote an earlier version of this chapter [1]. Together they made novel use of radionuclide‐labeled microspheres to quantitatively study cardiac output and organ blood flow in the undisturbed, conscious fetus, typically using the fetal lamb model [2–4]. These techniques, with variations, remain widely used for in vivo measurement of total and regional perfusion. In a normal adult blood flow is in series; that is, a sequential flow of blood from the systemic venous system to the right heart, to the lungs, and to the left heart, where well‐oxygenated blood is eventually pumped back into the systemic circulation. Fetal circulation is in parallel, where the right and left hearts control different proportions of the total blood volume. The total fetal cardiac output is thus a sum of both ventricles’ output – the combined ventricular output (CVO) or the biventricular output. The general pattern favors the streaming of well‐oxygenated blood from the placenta to the heart and the brain; and the less oxygenated blood streams into the umbilical arteries and, finally, back to the placenta. A number of elegant studies, including more recently with bold imaging using magnetic resonance imaging, have shown this differential blood oxygen content in different regions of the fetal circulation [5]. Nevertheless, the fetus at best lives in a hypoxic environment and a number of genes and molecular processes based on hypoxia‐inducible factor (1 and 2) have been shown to be affected by the relative hypoxia (e.g., expression of Erythropoietin gene in the fetal liver). How altered anatomy (e.g., transposition of great arteries) impacts the differential tissue oxygen delivery and consequences for altered biology (e.g., less oxygenated blood going to the brain or the myocardium instead) is under investigation. Three shunts that are critical to carrying out streaming of blood in the fetal circulatory system are (i) the ductus venosus (DV), (ii) the foramen ovale (FO), and (iii) the patent arterial duct (Figure 3.1). About half of the oxygenated placental blood returning via the umbilical vein (UV) is diverted directly into the inferior caval vein (IVC) via the DV, thus bypassing the liver parenchyma [4]. When this well‐oxygenated blood reaches the right atrium (RA) it is preferentially shunted across the atrial septum to the left atrium (LA) via the FO, thus making its way via the left ventricle (LV) to the coronary and cerebral circulations. The deoxygenated venous return from these circulations and the upper body drains via the superior caval vein (SVC) into the RA, streaming directly into the right ventricle (RV) with almost no blood traversing the FO thus minimizing mixing with the oxygenated blood going from the IVC to the LA [4]. The RV then pumps this relatively deoxygenated blood into the main pulmonary artery (PA), the majority of it flowing into the descending aorta via the arterial duct and from there supplying the abdominal viscera, the lower body, and the umbilical arteries, with reoxygenation occurring at the placenta. The lower‐body venous return is via the IVC to the RA, being split between the shunt across the FO and the normal flow via the tricuspid valve to the RV [6]. Figure 3.2 illustrates flows in the main vessels, shunts, and chambers as a proportion of the biventricular output. Note that the lungs receive minimal flow in the fetus, but quickly transition to receive the full cardiac output in the neonate. Figure 3.1 Diagrammatic representation of normal fetal circulation, major flow patterns, and blood hemoglobin oxygen saturations. AAo, ascending aorta; DAo, descending aorta; DV, ductus venosus; FO, foramen ovale; IVC, inferior caval vein; LA, left atrium; LHV, left hepatic vein; LPA, left pulmonary artery; LPV, left pulmonary veins; LV, left ventricle; MPT, main pulmonary trunk; P, placenta; PS, portal sinus; PV, portal vein; RA, right atrium; RHV, right hepatic vein; RPA, right pulmonary artery; RPV, right pulmonary veins; RV, right ventricle; UA, umbilical arteries; UV, umbilical vein. Source: Mavroudis C, Backer CL 2003 / with permission of Elsevier. The richly oxygenated UV blood splits in half as it enters the liver, being distributed equally between the DV and the liver parenchyma [7]. The DV blood flows into the suprahepatic IVC, streaming dorsal and leftward to that of the blood returning from the lower body, facilitating its preferential shunting across the FO and into the LA and, hence, to the brain and myocardium [8]. The other half of UV blood flow enters the liver preferentially via the portal sinus and left portal vein, representing about 80% of total hepatic blood flow. The remaining 20% of hepatic blood flow arrives from the portal vein (15%) and hepatic arteries (5%) [7]. Figure 3.2 Representative values for percentages of fetal cardiac output (combined ventricular output) returning to and leaving the heart in normal fetal lambs. AAo, ascending aorta; DAo, descending aorta; DV, ductus venosus; FO, foramen ovale; IVC, inferior caval vein; LA, left atrium; LHV, left hepatic vein; LPA, left pulmonary artery; LPV, left pulmonary veins; LV, left ventricle; MPT, main pulmonary trunk; P, placenta; PS, portal sinus; PV, portal vein; RA, right atrium; RHV, right hepatic vein; RPA, right pulmonary artery; RPV, right pulmonary veins; RV, right ventricle; UA, umbilical arteries; UV, umbilical vein. Source: Mavroudis C, Backer CL 2003 / with permission of Elsevier. The crista dividens, the lower border of the septum secundum, forms the cephalad margin of the FO lying to the right of the atrial septum, overriding the IVC orifice. It splits the IVC stream into an anterior and rightward portion flowing into the RA and a posterior and leftward stream that flows into the LA. The latter stream is predominantly composed of the UV return that flowed via the DV. Some mixing does occur, in that some DV blood flows into the RA and some deoxygenated IVC blood flows into the LA, but the net result is that the left atrium has a significantly higher oxygen saturation than the right (Figure 3.1). Blood returning to the heart via the SVC is preferentially streamed into the tricuspid orifice by the crista interveniens, situated in the posterolateral wall of the RA. The coronary sinus flow is also directed toward the tricuspid valve and, hence, the RV. The pulmonary venous return is to the LA, mixing with blood crossing the FO. The RV is the dominant ventricle in the fetus. It predominantly perfuses the lower body, the abdominal organs, and the placenta. As illustrated in Figure 3.2, the RV contributes approximately 65% of CVO, while the LV contributes only 35% [9]. The RV output is composed of venous return from the SVC, coronary sinus, and the predominantly lower‐body return from the IVC, and is pumped mostly to the descending aorta via the arterial duct, with only 8% of the RV output reaching the lungs. This preferentially distributes deoxygenated venous return to the placenta for reoxygenation. With progression of gestation, the relative proportion of blood that travels to the fetal lungs increases and by the third trimester it is much greater than previously thought. There are also clinical case reports now of increasing this blood flow through maternal hyperoxygenation, which can serve as both therapeutic means as well as diagnostic (e.g., to assess pulmonary venous obstruction in response to increased pulmonary blood flow). The latter findings suggest that the third trimester is key in the development of the pulmonary vasculature, relevant to the care of fetuses with intact atrial septum. While the LV pumps a relatively small percentage of the total CVO, it serves to preferentially deliver the oxygen‐enriched blood from the UV and FO to the brain and myocardium. Blood streaming into the LV has a relatively high oxygen saturation of 60% and perfuses the ascending aorta, resulting in 21% of the CVO reaching the brain and upper body via the arch branches, 4% reaching the coronary arteries, and 10% reaching the descending aorta via the isthmus. Hence, some saturated blood from the LV entering the descending aorta may perfuse the placenta, contributing to the physiological “left‐to‐right” shunt equivalent in the fetus [10]. Individual organ blood flows are shown in Figure 3.3 [4] as proportions of the CVO. Figure 3.3 Average combined cardiac output distributed to different organs or parts in eight different fetal animals. Source: Adapted from [4]. Table 3.1 Normal fetal pH and blood gas data. Source: Heymann 2003 / with permission of Elsevier. Table 3.1 shows some representative fetal blood gas values. Although the systemic arterial partial pressure of oxygen (pO2) in the fetus is low, hemoglobin O2 saturation is higher in the fetus than the adult because of fetal hemoglobin and the consequent left shift of the oxyhemoglobin dissociation curve. Fetal O2 consumption is significantly less, as the work of breathing is absent and thermoregulation requirements in utero are far less [11]. Fetal vascular pressures (shown in Figure 3.4) also reflect the blood streaming patterns described previously. The high flow from the placenta results in the UV having a 3–5 mmHg higher pressure than the IVC. The RA pressure is higher than the LA and, along with the kinetic energy of blood streaming via the IVC, contributes to the shunting of blood across the FO [6]. The arterial duct offers little resistance to flow and pressures in the RV and the main pulmonary trunk are only slightly higher (1–2 mmHg) than in the aorta and the LV. High resistance in the pulmonary vascular bed, in contrast, serves to limit blood flow through the fetal lungs and also directs blood to the systemic circulation via the arterial duct. Figure 3.4 Representative values for vascular pressures in normal fetal lambs. AAo, ascending aorta; DAo, descending aorta; DV, ductus venosus; FO, foramen ovale; IVC, inferior caval vein; LA, left atrium; LHV, left hepatic vein; LPA, left pulmonary artery; LPV, left pulmonary veins; LV, left ventricle; MPT, main pulmonary trunk; P, placenta; PS, portal sinus; PV, portal vein; RA, right atrium; RHV, right hepatic vein; RPA, right pulmonary artery; RPV, right pulmonary veins; RV, right ventricle; UA, umbilical arteries; UV, umbilical vein. Source: Mavroudis C, Backer CL 2003 / with permission of Elsevier. At birth, the pattern of circulation changes from the fetal “parallel” type to the adult “series” type, leading to more efficient oxygen uptake and delivery [12]. This happens with rapid establishment of pulmonary circulation, loss of placental circulation, and the closure of the ductus vein, the FO, and eventually the arterial duct. There is a rapid increase in O2 consumption, probably related to the work of breathing and thermoregulation, with an increase in cardiac output, a decrease in pulmonary vascular resistance, and an increase in pulmonary blood flow. The consequent increase in pulmonary venous return to the LA coupled with a decrease in IVC flow to the RA from cessation of placental UV return reverses the pressure difference between the LA and the RA, closing the flap valve of the FO. Pressures are also reversed across the ductus arteriosus (DA), with a left‐to‐right flow reversal being established before it closes. In the fetus, the patency of the arterial duct is maintained by prostaglandin E2 (PGE2) and prostacyclin (PGI2) [13]. The initial functional closure that occurs within 12 hours of birth is thought to be mediated by the increase in systemic pO2, though other vasoactive substances may also contribute [14]. A reduction in circulating prostaglandin levels is implicated in persistent ductal closure. Eventually there is fibrous replacement of the musculature of the arterial duct with formation of the arterial ligament. The high fetal pulmonary vascular resistance (PVR) is attributed to vasoconstriction in the thick medial layer of the small branches [15]. With increasing gestation there is growth of new arteries, an increase in the cross‐sectional area of the vascular bed, a drop in the PVR, and a slight increase in pulmonary blood flow [15]. Hypoxia is a major vasoconstrictive factor, as are acidosis and the leukotrienes [16, 17]. A diverse group of vasoactive substances cause fetal and perinatal pulmonary vasodilatation – bradykinin, histamine, acetylcholine, PGE1, PGE2, prostaglandin D2 (PGD2), PGI2, and catecholamines, to name a few. Their effects are mediated by the endothelial release of secondary substances like nitric oxide (NO). A baseline relaxation of the fetal pulmonary vasculature has been attributed to NO [18]. Ventilation causes a mechanical stretch‐induced production of PGI2 in the fetal lung, with a drop in PVR that is accentuated with oxygen [19]. A calcium‐dependent potassium channel activation has been hypothesized to mediate this effect of oxygen [20]. At birth, a combination of ventilatory stretch, increased alveolar oxygen tension, local NO, and PGI2 production blend to produce a dramatic fall in PVR and a resultant striking increase in pulmonary blood flow. A role for ventilation‐induced local mast cell degranulation and release of histamine and PGD2 has also been suggested in contributing to postnatal pulmonary vasodilation [21]. The immature fetal myocardium displays significant structural and hence functional differences from that of the adult. Not only are myocardial cells smaller, smooth, and rounded as opposed to being rod‐shaped as in the adult, the cytoplasm has proportionately more water and lower myofibril content and myocardial cells are not all arranged parallel to the cellular axis. This may account for the differences in force generation and in the fetal myocardium being stiffer and less compliant. The Frank–Starling mechanism is intact in the fetus, at least at low atrial pressures, but studies have differed on ventricular performance at higher atrial filling pressures [22–25]. Increases in arterial pressure significantly depress cardiac function in the fetus [25]. It does appear that in the fetus the preload and afterload are interrelated. The enhancement in stroke volume occurring after increasing the filling pressure by volume loading is stifled by a simultaneous augmentation in the afterload. It remains a matter of great interest how the neonatal LV transitions from a low‐pressure, low‐output chamber to functioning at systemic pressures controlling a full cardiac output abruptly at birth. Changes in contractility, increase in beta adrenergic receptor density, and postnatal thyroid hormone changes have been suggested to aid in this transition [26, 27]. The utility of fetal cardiac intervention is based on the fundamental principle that congenital heart disease evolves in utero, and that intervention in the fetus may beneficially alter the natural progression of the disease [28, 29]. From a historical perspective, the concept of fetal intervention stemmed from early attempts to treat fetal erythroblastosis by transfusion of red blood cells into the abdomen of the fetus. Remarkably, this crude technique worked and was the impetus for the early attempts at intervention. Subsequently others, in particular Dr. Michael Harrison, considered by many as the father of fetal surgery, extended the concept of fetal intervention to noncardiac lesions such as congenital diaphragmatic hernia and other lesions where in utero injury from unfettered pathophysiology could be altered [30]. Today, treatment of myelomeningocele is an accepted intervention at a number of select centers and still other unique pathologies are being addressed by both minimally invasive as well as open fetal surgery. From the cardiac standpoint, fetal cardiac intervention similarly evolved from the notion that hemodynamic effects of certain congenital lesions may cause progression in utero to ventricular hypoplasia, or to myocardial injury leading to congestive heart failure, arrhythmias, or fetal hydrops. Fetal pulmonary and neurologic development may also be affected [29, 31]. Such in utero progression has become more evident through intentional study of the natural history of congenital lesions throughout gestation, which in turn has been enabled through advances in fetal cardiac imaging. Detrimental progression of structural heart disease in utero has been demonstrated for several lesions, including critical valvar aortic stenosis, obstructive left heart lesions with intact atrial septum, and critical valvar pulmonary stenosis or pulmonary atresia. In each of these lesions, fetal intervention may theoretically alleviate flow restriction enough to limit its progression in utero, thereby reducing morbidity at birth and facilitating further surgical care. Both surgical and catheter‐based techniques for fetal cardiac intervention have been proposed; however, closed heart interventional techniques have the greatest clinical promise and are the only methods in current clinical use in humans. Several decades of experimental work have been invested in the development of fetal open‐heart surgery; however, a number of important challenges have so far limited its clinical application. These include fetal bypass and its related placental dysfunction, fetal myocardial protection, postoperative fetal support, risk of pregnancy loss and preterm labor, and risk to the mother. Beginning in the 1970s, the group led by Drs Rudolph and Heymann pioneered early research into fetal cardiac surgery by creating fetal lamb models of congenital lesions, including banding of the ascending aorta to simulate stenosis and placement of a left atrial balloon to create inflow limitation [32]. Fetal lamb models of aortic and pulmonary banding and closed‐heart techniques for surgical repair were also reported [33, 34]. However, repair of fetal intracardiac lesions would require fetal cardiopulmonary bypass (CPB), into which extensive research followed, mostly using near‐term and earlier‐gestation lamb models as well as primate models.
CHAPTER 3
Fetal Cardiac Physiology and Fetal Cardiac Intervention
Physiology of the Fetal Circulation
History of Fetal Circulation Research
Fetal Circulation
Fetal Intra‐ and Extracardiac Shunts
Venous Return
Cardiac Output, Oxygenation, and Distribution
Umbilical
Descending
Ascending
Value
vein
aorta
aorta
pH
7.40–7.45
7.35–7.40
7.37–7.42
PO2 (mmHg)
28–33
20–24
22–26
PCO2 (mmHg)
45–50
48–53
46–51
Intracardiac and Vascular Pressures
Circulatory Changes at Birth
The Transition
Closure of the Arterial Duct
Pulmonary Circulation
Myocardial Performance
Fetal Cardiac Intervention
Rationale for Fetal Cardiac Intervention
Limitations for Success of Fetal Cardiac Surgery
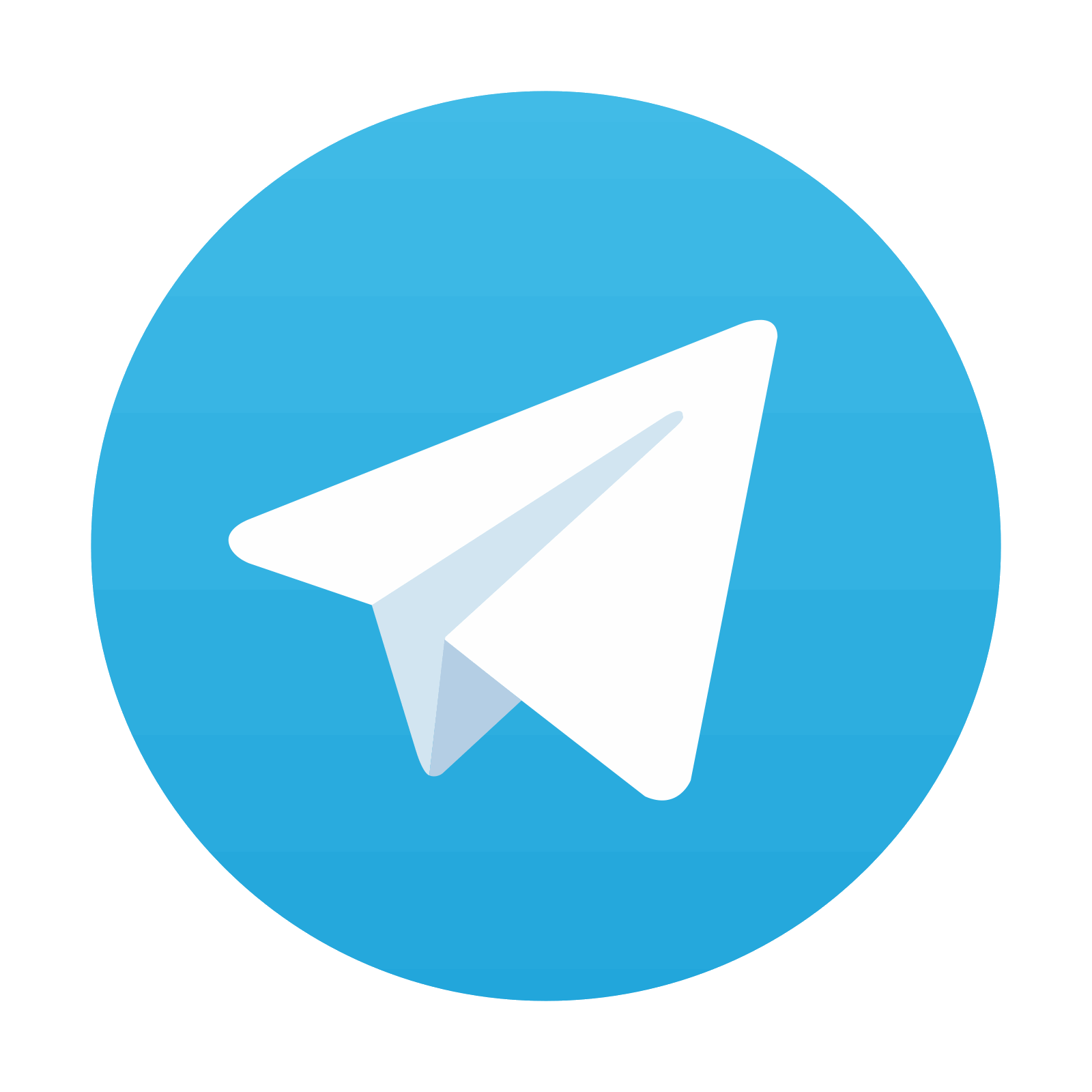
Stay updated, free articles. Join our Telegram channel
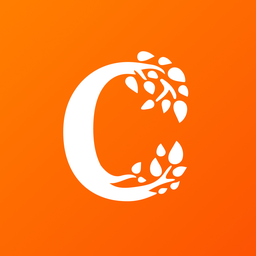
Full access? Get Clinical Tree
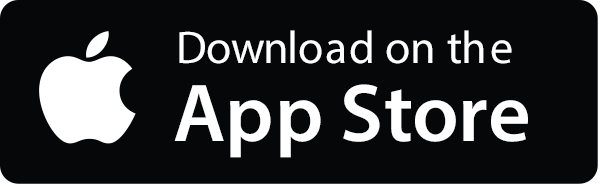
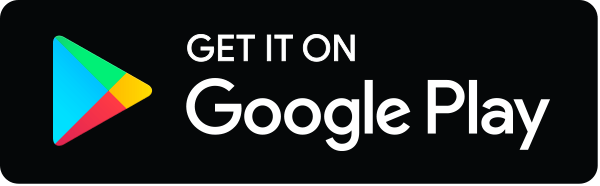