Decline in exercise performance Submaximal versus maximal exercise performance Relevance of the decline in maximum exercise capacity Central and peripheral fatigue and physical performance Hypoxic training for sea-level exercise Living-training hypoxic paradigms Timing of altitude training relative to competition Potential negative consequence of altitude training One of the most important consequences of going to high altitude is a reduction in both maximal aerobic capacity and endurance exercise performance. In this chapter, we examine the physiology of oxygen transfer from the air to the mitochondria in the face of the reduced inspired PO2, specifically as it pertains to exercise at high altitude. We review how the primary physiological responses to exercise are altered in a hypoxic environment and consider the physiological factors that contribute to the decline in maximum exercise capacity, including discussion of whether exercise performance is limited not only by factors related to oxygen transport, but also fatigue within the central and peripheral nervous system. Finally, we discuss the evidence base for various training paradigms whereby athletes attempt to utilize terrestrial or simulated high altitude exposure to improve athletic performance both at altitude and at sea level. The literature on exercise at altitude is very extensive, and the present chapter is necessarily selective. For a more detailed review on the physiology of exercise at high altitude the reader can refer to several more extensive monographs and reviews on the topic (Calbet et al. 2016; Casey and Joyner 2012; Hennis et al. 2015; Lovering et al. 2015; Stembridge et al. 2016; Sutton et al. 1987; Sutton et al. 1983; Verges et al. 2012; Wagner 1996b; Walsh and Oliver 2016). Two of the most noteworthy features of exercise upon acute exposure to high altitude are the increased perception of effort with exercise (dyspnea on exertion) and the decrease in maximum exercise capacity. Regardless of the baseline level of fitness, following ascent to high altitude, the majority of individuals report increased dyspnea (or shortness of breath) with exercise that resolves quickly with rest. This tends to be present during the first several days at a given elevation, subsides with acclimatization, but returns again upon ascent to higher elevation. Numerous studies since the early work of Zuntz et al. (1906) have demonstrated that maximum exercise capacity, or maximal oxygen consumption ( Figure 18.1 Further data on the reduction in V.O2max with ascent came from studies done as part of the 1981 American Medical Research Expedition to Everest (AMREE). Here, progressive cycling tests were performed at an altitude of 6300 m with subjects breathing ambient air, as well as reduced oxygen gas mixtures (FIO2 of 0.16 and 0.14) simulating higher elevations (West et al. 1983a). The lowest FIO2 in these studies yielded an inspired PO2 of 42.5 mmHg, which is roughly equivalent to that experienced on the summit of Mt. Everest. As demonstrated in Figure 18.2, Figure 18.2Oxygen uptake plotted against work rate during the 1981 American Medical Research Expedition to Everest. Closed circles (•): sea level; Closed triangles (▴): 6300 m breathing ambient air; Open circles (⚬): 6300 m, FIO2 0.16; Open triangles (△): 6300 m, FIO2 0.14. (Source: West et al. 1983a.) Figure 18.3Maximal oxygen uptake plotted against inspired PO2 from the 1981 American Medical Research Expedition to Everest (AMREE, △) and those reported by Pugh et al. (Pugh 1964a) from the 1961 Silver Hut Expedition and additional measurements on Mount Everest (⦁). 1 torr = 1 mmHg. (Source: West et al. 1983a.) One of the noteworthy features of the data from Cerretelli mentioned previously, was that the maximum exercise capacity data did not show any consistent differences between subjects exposed to acute hypoxia and those who have had the advantage of acclimatization. In other words, unlike some other aspects of the physiologic responses to high altitude, such as arterial oxygen content or resting cardiac output, Figure 18.4Decline in The importance of the fall in Figure 18.5Illustration of an individual cycling at a power output of 200 W, which requires an oxygen uptake of 2 L/min, a value around 50% of sea level The observation from Cerretelli that acclimatization does not lead to improvements in Although the decline in Despite a considerable amount of research into this question, the precise mechanism for the decline in Figure 18.6The oxygen transport cascade from the atmosphere to mitochondria within skeletal muscle. (Image courtesy of Justin Lawley.) Exercise at high altitude is accompanied by very high levels of ventilation. Indeed, this was one of the most obvious features of climbing at extreme altitudes noted in the early Everest expeditions (Pugh 1957). Ventilation is normally expressed at body temperature, ambient pressure, and with the gas saturated with water vapor (BTPS). This is because the volumes of gas moved then correspond to the volume excursions of the chest and lungs. Ventilation can also be expressed at standard temperature and pressure for dry gas (STPD). These volumes are very much smaller at high altitude and bear no obvious relationship to the actual chest movements. However, the oxygen uptake and carbon dioxide output are traditionally expressed in these units so that the values are independent of altitude. For a given work level, the ventilation expressed as BTPS is higher at high altitude than at low elevation. Figure 18.7 shows data obtained during the 1960–61 Silver Hut Expedition with ventilation expressed as both BTPS and STPD (Pugh 1964b). With the latter, the values also tend to be somewhat higher than those measured at sea level, especially at work levels approaching the maximum for the altitude, but the differences are clearly much less than when ventilation is expressed as BTPS. Figure 18.7Relationship between ventilation, both BTPS and (a) STPD (b), and oxygen uptake at various altitudes. (Source: Pugh 1964b.) Ventilation (BTPS) can reach extremely high levels, as evidenced by data obtained during AMREE at an altitude of 6300 m (PB 351 mmHg). In eight subjects who exercised at a work rate of 1200 kg min−1, the mean ventilation (BTPS) was 207 L min−1 with a mean respiratory frequency of 62 breaths min−1. These values were for a mean oxygen consumption of 2.31 L min−1 and correspond to a ventilatory equivalent ( With climbing at high altitude, there is a trade-off between the energy output required by the muscles of locomotion and that required by the muscles of respiration. Cibella et al. (1999) demonstrated in subjects that, in spite of the lower gas density, there was substantially greater energy expenditure for respiration at high altitude (26%) than at sea level (5.5%), theoretically depriving the locomotory muscles of perfusion. Marciniuk et al. (1994) have shown that it is primarily the diaphragm that competes for the limited blood flow. At sea level, increasing the work of breathing does increase muscle sympathetic nerve activity and leg vascular resistance and decrease leg blood during exercise (Babcock et al. 1995; Barclay 1986; Dominelli et al. 2019; Harms et al. 1997). At high altitude, this process is thought to be more influential as ventilation is much greater. Certainly, at sea level in acute hypoxia, elevations in sympathetic vasoconstrictor activity emanating from both limb and respiratory muscle metaboreceptors during exercise seem to constrain blood flow and oxygen transport, thereby promoting fatigue of both sets of muscles (Sheel et al. 2018). This has been demonstrated in studies by Harms et al. (1997) in which leg blood flow declined as the work of breathing—manipulated through the use of a proportional assist ventilator—increased. Broadly consistent with these observations, Lundby et al. (2006) convincingly demonstrated a persistent decrease in blood flow to the lower extremities in lowlanders after eight weeks of acclimatization to 4100 m, suggesting this pattern may be an important factor in limiting maximal exercise at very high altitudes. Part of this decrease in blood flow can be restored in this same population when hemoglobin and presumed blood viscosity are decreased with isovolemic hemodilution (Calbet et al. 2002). The increase in the work of breathing is mitigated to some extent by the lowered density of the air at high altitude. This is demonstrated by the fact that maximal voluntary ventilation is increased at high altitude. For example, Cotes (1954) showed that the maximal voluntary ventilation (BTPS) increased from 158 at sea level to 197 L min−1 at a simulated altitude of 5180 m in a low-pressure chamber. In a further study, a mean value of 203 L min–1 was observed at a simulated altitude of 8250 m (Cotes 1954). It is interesting that these extremely high levels of ventilation are not seen at the highest altitudes. For example, when two subjects on AMREE breathed an oxygen mixture with an FIO2 of 0.14 at an altitude of 6300 m (PIO2 ∼42.5 mmHg) to simulate the summit of Everest, the maximal exercise ventilation was only 162 L min−1. A similar pattern of ventilatory responses had been seen during the 1960–61 Silver Hut Expedition, when maximal exercise ventilation had a mean value of 173 L min−1 at 5800 m, 161 L min−1 at 6400 m, and only 122 L min−1 at the equivalent of 7400 m. A reasonable explanation for the lower exercise ventilation seen in both of these studies is that maximum work rates achieved were markedly lower with increasing elevation. For example, in the Silver Hut expedition, Interestingly, as illustrated in Figure 18.8, ventilation does not return to the sea-level values with administration of 100% oxygen, but is intermediate between the high altitude and sea level values for ambient air. This observation suggests some oxygen-independent effect, perhaps due to the increased sensitivity of the carotid body, the primary organ of ventilatory acclimatization. Changes in the sensitivity of the carotid body are discussed in Chapter 9. Figure 18.8Effect of breathing oxygen at sea-level pressure on ventilation and heart rate in acclimatized subjects at 5800 m. The points are mean values from two subjects. (Source: Pugh et al. 1964.) The pattern of breathing during exercise at high altitude is characterized by very high frequencies and relatively small tidal volumes. The highest measurements of respiratory frequency and tidal volume yet made were those on Chris Pizzo during AMREE (West et al. 1983b). He climbed for about seven minutes at an altitude of 8300 m (PB 271 mmHg). During the middle four minutes of this period, his mean respiratory frequency was 86 ± 2.8 (SD) breaths per minute, mean tidal volume was 1.26 L, and mean ventilation was 107 L min−1 at BTPS. Thus, his breathing was shallow and extremely rapid. Unlike at sea level where arterial PO2 and oxygen saturation remain constant with progressive exercise in the majority of healthy individuals, the arterial PO2 and oxygen saturation decrease with progressive exercise at high altitude (Figure 18.9). Several factors likely contribute to this phenomenon. Figure 18.9Arterial oxygen saturation as measured by ear oximetry plotted against work rate at sea level and 6300 m altitude. The two lower lines were obtained with subjects breathing an FIO2 of 0.16 and 0.14 at 6300 m. Note the fall in oxygen saturation with increasing work rate at high altitude. (Source: West et al. 1983a.) For many years, it was believed that the only change in ventilation–perfusion relationships at high altitude was a more uniform topographical distribution of blood flow due to the increased pulmonary arterial pressure resulting from hypoxic pulmonary vasoconstriction (HPV) (Chapter 11). For example, measurements made with radioactive xenon have shown that the topographical differences of blood flow between the apex and base of the upright lung are reduced at an altitude of 3100 m (Dawson 1972). As discussed in Chapter 8, measurements by Wagner and his co-workers (Wagner 1988) show a broadening of the distribution of ventilation–perfusion ratios during high levels of hypoxic exercise, potentially mediated via hypoxic pulmonary vasoconstriction and other factors such as changes in blood viscosity and sympathetic activity (see Chapter 8, Figure 8.12). This change in the distribution of ventilation and perfusion is marked by an increase in blood flow to poorly ventilated lung units, as demonstrated in healthy subjects who are exercising while acutely exposed to hypoxia in a low pressure chamber (Gale et al. 1985), healthy subjects who are exercising while inhaling low oxygen mixtures (Hammond et al. 1986), and healthy subjects during a 40-day exposure to low barometric pressure in a chamber during Operation Everest II (Wagner et al. 1987). Evidence from this latter study suggests that the ventilation-perfusion abnormalities are most likely to be seen in poorly acclimatized subjects following a rapid ascent, with the most marked abnormalities seen with the most severe levels of hypoxia, and at the heaviest levels of exercise. Diffusion limitation of oxygen transfer in the lung is the primary reason for the consistent fall in the arterial PO2 and oxygen saturation during exercise at high altitude and is one of the chief limiting factors for maximal exercise at extremely high elevations (Chapter 19). In contrast to the resting state, there is tenuous evidence that the diffusing capacity of the blood-gas barrier changes with acclimatization to high altitude in lowland subjects (Dehnert et al. 2010; Mairbaurl et al. 2019; Martinot et al. 2013). Measurements from the 1960–61 Silver Hut Expedition showed that the diffusing capacity of the blood-gas barrier for a given level of exercise was the same as at sea level (West et al. 1962). Overall pulmonary diffusing capacity for carbon monoxide increased by 19% at an altitude of 5800 m, but this could be attributed to the more rapid rate of the combination of carbon monoxide with oxygen because of the low prevailing PO2. The volume of blood in the pulmonary capillaries as determined by measuring the diffusing capacity at two values of alveolar PO2 showed no change or possibly a slight fall. This may have been due to hypoxic pulmonary vasoconstriction. These results also imply that, in acclimatized subjects, the transit time for red cells in the pulmonary capillaries at a given work level is approximately the same as at sea level. The transit time of the pulmonary capillary blood is given by the pulmonary capillary blood volume divided by the cardiac output (Roughton 1945). As discussed later, there is good evidence that in acclimatized lowlanders at high altitude, the cardiac output for a given work level is the same as at sea level (Pugh 1964a; Reeves et al. 1987; Wagner 2000). Thus, since both the pulmonary capillary blood volume and the cardiac output are essentially unchanged, this indicates that the transit time through the pulmonary capillaries will also be the same as at sea level; however, because of the lower driving pressure for oxygen from the alveolus to the blood, there is still not enough time for equilibration with the pulmonary capillary blood. Calbet et al. (2008) furthered this discussion by showing that at moderate altitude during steady-state exercise (100–120 watts) with a cardiac output of ∼2 L min−1, there was no widening of the alveolar-arterial oxygen difference ([A−a]ΔO2). Even at this altitude and level of work, the model of Piiper and Scheid (1980) would have predicted some diffusion limitation. It is generally accepted though that the magnitude of the widening of the (A−a)ΔO2 and diffusion limitation is proportional to the altitude and cardiac output. Lovering et al. (2008) postulated an alternative mechanism for high altitude exercise hypoxemia beyond diffusion impairment. Using saline contrast echocardiography, they studied nine males during incremental exercise to exhaustion during normoxia and hypoxia (FIO2 = 0.12). Their intent was to evaluate shunting through intrapulmonary arteriovenous anastomoses induced by exercise and/or hypoxia. At rest, none of the subjects under normoxia and three of the subjects during hypoxia demonstrated a right-to-left shunt, whereas, during exercise, eight out of nine demonstrated right-to-left shunt in normoxia and nine out of nine demonstrated this in hypoxia. They hypothesized that increased pressures and/or blood flow opened anatomically defined microvascular channels, which created the right-to-left intrapulmonary shunt. While appealing on the surface, this proposed explanation has been challenged on several grounds (Hopkins et al. 2009). First, while the detection of shunt depends on the appearance of bubbles in the left atrium, the size of bubbles that transit the pulmonary vasculature is not known, nor is the importance of reaggregation of these bubbles on the left side. Second, previous quantitation of shunt by other methods has shown that, if there is any shunt at all, it is trivial and physiologically insignificant. For example, using the multiple inert gas elimination technique (MIGET), which elegantly quantitates pulmonary gas exchange between ventilation-perfusion inequality, shunt, and diffusion impairment at high levels of exercise, the percent that shunt might contribute to the impairment in gas exchange has never been shown to be >1.0%. Lovering et al. (2009) defended their findings by invoking precapillary gas exchange that could affect the transit of soluble gases across the alveolar-capillary membrane and thus underestimate shunt using the MIGET technique. Another potential source of right-to-left shunt is through a patent foramen ovale (PFO) (Lovering et al. 2016), which can be detected in ∼25–35% of the population (Elliott et al. 2013). Acute hypoxia is associated with an increased incidence of PFO during exercise (Imray et al. 2008; Lovering et al. 2008). However, short (Imray et al. 2008) or prolonged (Elliott et al. 2015) periods of acclimatization have seemingly little effect on exercising (A−a)ΔO2 or SpO2. Thus, while these anatomic shunts may be real, their physiologic significance remains unclear. On the basis of well-controlled animal studies, it has been proposed that contrast echocardiography is sensitive, but not specific, often detecting small anatomical shunts which are inconsequential for gas exchange (Stickland et al. 2019). This issue is discussed further in Chapter 19. Further work will be necessary to clarify these issues and their relevance at high altitude. The arterial PO2 and PCO2 are both reduced with exercise at high altitude. Arterial PO2 typically falls further on exercise because of diffusion limitation and the other factors noted above, while at high work levels, the arterial PCO2 often falls below the resting value because alveolar ventilation increases more than carbon dioxide production (i.e., hyperventilation). The marked increase in ventilation and carbon dioxide elimination is associated with an increased respiratory exchange ratio, which may increase to values above 1.2 at the highest workloads at very high altitudes (West et al. 1983b). The implications of such shifts in the respiratory exchange ratio on fuel oxidation and metabolism are outlined in Chapter 15. The next step in the oxygen cascade is the oxygen-carrying capacity of arterial blood. As red cell volume is a predictor of The next critical step in the oxygen cascade is oxygen transport via cardiac output ( In nonacclimatized and poorly acclimatized lowlanders who go acutely to high altitude, heart rate and cardiac output are increased during exercise for a given work level compared with sea-level values. These responses are seen at sea level following inhalation of low oxygen mixtures, and on acute exposure to high altitude (Asmussen and Consolazio 1941; Honig and Tenney 1957; Keys et al. 1943; Kontos et al. 1967; Stenberg et al. 1966; Vogel and Harris 1967). In well-acclimatized lowlanders at high altitude, the relationship between cardiac output and work rate returns to the sea-level value (Pugh 1964a; Reeves et al. 1987) with the maintenance of contractile function (Reeves et al. 1987). For example, Pugh (1964a) showed this relationship during the Silver Hut expedition at an altitude of 5800 m where the measurements were made by the acetylene rebreathing technique (Figure 18.10). Further measurements were made by Cerretelli (1976) at the Everest base camp where the subjects had acclimatized for two to three months as well as during Operations Everest II (Reeves et al. 1987) and Operations Everest III (Boussuges et al. 2000) (Figure 18.11). Figure 18.10Cardiac output in relation to work rate and oxygen uptake as obtained from four well-acclimatized subjects during the Silver Hut expedition. Note that the cardiac output/work rate relationship is the same at an altitude of 5800 m (×, barometric pressure 380 mmHg) as at sea level (•). (Source: Pugh 1964a.) Figure 18.11Cardiac output, measured by thermodilution, and stroke volume plotted against oxygen uptake ( Maximal heart rate, that is the heart rate at maximal exercise, is reduced at high altitude (Lundby et al. 2001a; Lundby et al. 2006; Lundby and Hall 2001; Mourot 2018) (Figure 18.8). For example, in Operation Everest II, maximal heart rate decreased from 160 ± 7 at sea level to 137 ± 4 at a simulated altitude of 6100 m, 123 ± 6 at 7620 m, and 118 ± 3 at 8848 m (Reeves et al. 1987). For a given work level, heart rates were greater at high altitude compared with sea level, though, interestingly, there seemed to be little difference between the measurements made at barometric pressures of 347 mmHg, 282 mmHg, and 240 mmHg, as shown in Figure 18.12. This is possibly a reflection of the limited degree of acclimatization of the subjects at the highest altitudes (West 1988). The difference between field and chamber studies was emphasized by Lundby and Hall (2001), who showed that although peak heart rate was reduced at an altitude of 8750 m on Mount Everest, the heart rates were higher than found in chamber experiments. In Operation Everest III (Comex ’97), maximal heart rates were also reduced compared with sea-level values (Richalet et al. 1999). Figure 18.12Regression lines for heart rate versus oxygen uptake at barometric pressures of 760 mmHg, 347 mmHg, 282 mmHg, and 240 mmHg during Operation Everest II. For the measurements at 240 mmHg, the subjects breathed an oxygen mixture to give an inspired PO2 of 43 mmHg. (Source: Reeves et al. 1987.) Oxygen breathing in acclimatized subjects at high altitude reduces the heart rate for a given work level (Pugh et al. 1964). This is shown in Figure 18.8, where it can be seen that the heart rate for a given work level was actually lower than the corresponding measurements at sea level. A possible explanation for the reduction below the sea level value is the fact that the arterial PO2 at this altitude of 5800 m with 100% oxygen breathing is higher than at sea level, and also that these subjects had much higher hemoglobin levels than at sea level because of the high altitude polycythemia. With an elevated hemoglobin mass and thus oxygen-carrying capacity, convective oxygen transport and oxygen delivery can be maintained and a lower heart rate (i.e., cardiac output) both at rest and during exercise (Stembridge et al. 2019b). The role of the autonomic nervous system in controlling heart rate, as well as cardiac output, during exercise is well established. Exposure to hypoxia increases plasma concentrations of epinephrine and norepinephrine (Hopkins et al. 2003) and muscle sympathetic nerve activity during exercise (Katayama et al. 2011). Thus, it has been argued that the reduction of maximal heart rate in acclimatized subjects represents a physiological adaptation that reduces cardiac work under conditions of limited oxygen availability (Richalet 1990). There is good evidence that hypoxia induces downregulation of β-adrenergic receptors in animal and human hearts (Kacimi et al. 1992; Mazzeo and Reeves 2003; Richalet et al. 1988; Voelkel et al. 1981). Yet, the heart rate response to hypoxic exercise mirrors the sea level condition despite β-adrenergic blockade (Bogaard et al. 2002; Boushel et al. 2001; Hopkins et al. 2003). Inhibition of the parasympathetic nervous system via the muscarinic antagonist glycopyrrolate restores maximal heart rate to sea-level values (Boushel et al. 2001), suggesting that incomplete vagal withdrawal plays a major role in the reduced maximal heart rate. Interestingly, the heart rate response to exercise is still apparent, albeit at a reduced maximal heart rate, with combined inhibition of β-adrenergic and muscarinic receptors (Siebenmann et al. 2015) hinting that alternative mechanisms such as cardiac α-adrenergic transmission may be involved. While, theoretically, dopamine may act to blunt noradrenaline release and affect heart rate, exercise in hypoxia does not consistently increase the concentration of circulating dopamine (Hopkins et al. 2003), nor are dopamine D2 receptors involved in the hypoxia-induced decrease in maximal heart rate (Lundby et al. 2001b). As explained previously, contractile function of the heart is maintained on ascent to high altitude due to preservation of the left ventricular pressure-volume relationship (Reeves et al. 1987). In fact, because resting left ventricular end-diastolic volume is reduced at altitude, systolic function may even be improved, as left ventricular end-systolic volume is smaller at altitude than at sea level (Fowles and Hultgren 1983). These data are also supported by normal or slightly better indices of myocardial function via echocardiography (Stembridge et al. 2015). The fact that the cardiac output response to work rate is the same as at sea level while heart rate remains high after several weeks of acclimatization implies that stroke volume during exercise is reduced. A possible contributing factor is a fall in plasma volume. Yet, while initial observations showed that plasma volume expansion improves For a given work rate, pulmonary artery pressure is increased during exercise at altitude compared with sea level in both unacclimatized (Kronenberg et al. 1971) and acclimatized (Groves et al. 1987) lowlanders. For example, Hergistad and Robbins (2009) exposed 10 subjects to eight hours of hypoxia (end-tidal PO2 55 mmHg) and ambient air at low altitude and then used Doppler echocardiography to measure the maximum pressure gradient across the tricuspid valve to estimate pulmonary artery systolic pressure. The subjects were then studied breathing ambient air at rest and with exercise at a heart rate 30 beats per minute above resting values. There was a modest, but significant increase in the pressure gradient across the tricuspid valve at rest and a 35% increase during exercise after the hypoxic exposure. While this increase in pulmonary artery pressure is likely the result of hypoxic pulmonary vasoconstriction, there is some evidence to suggest that structural remodeling of the pulmonary vasculature with acclimatization may contribute as well. In Operation Everest II, for example, pulmonary artery pressure did not return to normal when 100% oxygen was breathed even though the subjects had been at high altitude only two or three weeks (Groves et al. 1987), a finding supported by more recent data from a field study in healthy volunteers trekking to Everest base camp (Luks et al. 2017). These data suggest some structural remodeling of the pulmonary arteries takes place over a relatively brief time of exposure to hypobaric hypoxia and may contribute to the observed pulmonary vascular responses along with hypoxic pulmonary vasoconstriction. It has been speculated that a portion of the decrease in exercise performance at high altitude is attributable to impaired right ventricular function (and thus cardiac stroke volume) secondary to the observed pulmonary vascular response and right ventricular afterload (Naeije 2011; Naeije et al. 2010), although others have questioned this relationship (Anholm and Foster 2011). One way to assess the role of pulmonary hypertension in limiting exercise is to study the effect of various pulmonary vasodilators on pulmonary artery pressure and exercise performance in hypoxia. To date, multiple studies have examined the effect of the phosphodiesterase-5 inhibitor (PDE-5), sildenafil. While the medication does lower pulmonary artery pressure during exercise, it has not been consistently shown to improve exercise capacity (Carter et al. 2019; Ghofrani et al. 2004; Richalet et al. 2005) (Figure 18.13). Figure 18.13Echocardiographic evaluation of pulmonary hemodynamics. Pulmonary artery pressure (PAP). *p <0.05 versus sea level pre; #p <0.05, ##p <0.01 sildenafil (filled squares) versus placebo (open circles); p <0.05, p <0.01 Day 1 versus sea level pre for the whole group; +p <0.05, +++p <0.001 sildenafil versus placebo for pooled high altitude with treatment values. (Source: Richalet et al. 2005.) Other studies have examined the role of endothelin receptor antagonists. For example, Faoro et al. (2009) used a double-blind randomized design and demonstrated that administration of Bosentan reduced pulmonary vascular resistance (PVR) and restored 30% of the hypoxia-induced decrease in V.O2max, while de Bissschop et al. (2012) demonstrated that sitaxentan reduced PVR and improved The last steps in the oxygen transport cascade involve delivery of oxygen to the exercising muscles and diffusion into the mitochondria. Studies at 4300 m and 5260 m have revealed that while oxygen saturation and red blood cell mass increase with acclimatization, net oxygen delivery to exercising muscles does not increase accordingly (Mazzeo 2008). This observed reduction in oxygen delivery seems to be the result of a 20–30% decrease in muscle blood flow during exercise, thereby offsetting the improvements in oxygen content. Consistent with these observations are the reported reductions in vascular conductance that are likely mediated via an increased vascular tone. Lundby et al. (2008), for example, infused adenosine triphosphate (ATP) (80 µg kg−1 min−1) during exercise in eight healthy male subjects at 4559 m and studied the hemodynamics and energetics of femoral artery blood flow during maximal exercise. Cardiac output was preserved while mean arterial pressure decreased. Although oxygen delivery was similar with and without ATP infusion, oxygen extraction decreased, and thus there was a 20% decrease in leg Beyond the alterations in blood flow, there has been considerable interest in the potential role of oxygen diffusion from capillaries to mitochondria in exercise at high altitude (Reeves 2004; Wagner 1996b). Wagner (1996b) analyzed the relationship between oxygen uptake and the PO2 of muscle capillary blood on the assumption that the uptake is limited by oxygen diffusion from the capillaries to the mitochondria (Hogan et al. 1988). Figure 18.14a shows a diagram relating oxygen uptake to the PO2 of muscle venous blood, taken as an index of muscle capillary PO2. The line sloping from top left to bottom right shows the amount of oxygen being delivered to the muscle by the capillaries (Fick principle). The line from bottom left to top right shows the pressure gradient for diffusion of oxygen from the red cells to the mitochondria (Fick’s law), assuming that the mitochondrial PO2 is nearly zero. The slope of this line is the lumped “diffusing capacity for oxygen” of the tissues. The point where the two diagonal lines cross represents the Figure 18.14(a) Diagram showing how Several pieces of evidence now support this concept. For example, a retrospective analysis of data from Operation Everest II showed that the points relating the PO2 of mixed venous blood to oxygen uptake tend to lie on a straight line passing through the origin (Wagner 2017). On the assumption that the PO2 of mixed venous blood reflects the PO2 of the blood in the capillaries of the exercising muscles, this relationship supports the notion. Indeed, it was this observation that prompted the hypothesis (Wagner 1988). More direct evidence comes from a prospective study in which normal subjects exercised at high workloads breathing hypoxic mixtures, and samples of femoral venous blood were taken via an indwelling catheter (Roca et al. 1989). Again, a plot of the PO2 of femoral venous blood against oxygen uptake for different inspired oxygen concentrations showed the points lying close to a straight line passing near to the origin. A similar plot was found when the calculated mean capillary PO2 was substituted for femoral venous PO2. Additional studies have been carried out on an isolated dog gastrocnemius preparation where the muscle was supplied with hypoxic blood and stimulated maximally. Again, a good relationship was found between the PO2 of the effluent blood and the maximal oxygen uptake at different levels of hypoxia (Hogan et al. 1988). This preparation allowed a test of two competing hypotheses, referred to above, and an alternative hypothesis that The diffusion-limitation hypothesis has also been tested in more recent studies. In one of these, the oxygen affinity of hemoglobin was increased by feeding dogs sodium cyanate, and it was shown that for the same convective oxygen delivery, the maximal oxygen concentration of dog muscle was reduced compared with animals in which the oxygen affinity was normal (Hogan et al. 1991). The converse experiment was also carried out by reducing the oxygen affinity of hemoglobin using the allosteric modifier methylpropionic acid. In this case, the dog muscle showed an increased maximal oxygen consumption at a constant blood oxygen delivery compared with an animal with a normal oxygen affinity of hemoglobin (Richardson et al. 1998). Animal studies indicate an increase in capillary density in some tissues as a result of chronic hypoxia. However, data available from human muscle biopsies indicate that while mRNA of VEGF is elevated with acute exposure to hypoxia (Horscroft et al. 2017) the number of capillaries remains constant in acclimatized lowlanders with no increase of mRNA expression of regulatory factors for angiogenesis (VEGF) during acclimatization (Horscroft et al. 2017; Lundby et al. 2004). On the other hand, the average distance over which oxygen diffuses is reduced because the muscle fibers become smaller, perhaps secondary to ongoing oxidative damage with prolonged high altitude exposure (Hoppeler et al. 1990; Lundby et al. 2004). There are changes in intracellular enzymes, such as an increase in muscle myoglobin, which may enhance oxygen diffusion. Mizuno et al. (2008) examined the effect of exercise versus sedentary existence at 5250 m or higher for 75 days. There was no difference on the previously noted effects of high altitude on muscle morphology or chemistry. In other words, muscle fiber size decreased; capillary number (2.1–2.2 capillaries per fiber) was not different between groups, while capillary density increased about 30%. Enzymes chosen to reflect mitochondrial capacity, citrate synthase and 3/hydroxyl-CoA-dehydrogenase, did not change after the altitude exposure, while muscle buffer capacity did increase with the altitude exposure, although not differently between the exercising and sedentary groups. Using high-resolution respirometry of skeletal muscle, Jacobs et al. (2013) also found unaltered mitochondrial function with acclimatization. Using 31P-NMR spectroscopy, Edwards (1936) studied trekkers to Everest Base Camp at 5300 m and climbers to 7950 m before and after the journey. Although the peak phosphate at rest was higher in the climbers prior to the trip, perhaps secondary to better conditioning, all of the other markers of mitochondrial function were preserved even after profound and prolonged altitude exposure. These findings confirm those from the earlier OEII chamber study (Green et al. 1989) that any changes in fiber area, capillary to fiber ratio and mitochondrial function seem independent of the level of physical activity at high altitude (Mizuno et al. 2008). Nonetheless, future studies in this area will be enhanced by precisely quantifying the amount of physical activity/training load that each individual undertakes at sea level and perform a matched training program during subsequent acclimatization to avoid person-specific (high versus low baseline fitness) detraining effects. While serum lactate can be elevated for many reasons, one of the more common causes is an imbalance between oxygen delivery and metabolic activity. Interestingly, during exercise at high altitude, blood lactate levels remain surprisingly low even following maximum exercise (Cerretelli 1980; Edwards 1936; Lundby et al. 2000; West 1986). Extensive observations that blood lactate is low in acclimatized subjects at high altitude, even during maximal work, were first made by Edwards (1936) during the 1935 International High Altitude Expedition to Chile, although Dill et al. (1931) had obtained some data prior to that. Figure 18.15 is redrawn from Edward’s paper and shows that the levels of blood lactate during exercise at altitudes up to 5340 m were essentially the same as at sea level. This means that the blood lactate levels for a given work level were apparently independent of tissue PO2. The only clear exceptions to this were the points shown by the open circles, which were obtained at the lowest altitude of 2810 m. The days spent at altitude are shown on the abscissa, and it is clear that in most instances these data were obtained before the subject had time to fully acclimatize. Since maximal work capacity declines markedly with increasing altitude, maximal blood lactate falls in acclimatized subjects as altitude increases. This issue is considered further in Chapter 19 and demonstrated in Figure 19.8. Figure 18.15Venous blood lactate after exercise as reported by Edwards from the 1935 International High Altitude Expedition to Chile. The lines are drawn through the sea-level values. In general, lactate levels at high altitude lie on the same line, the only obvious exceptions being measurements made at the lowest altitude of 2.81 km. The small figures above these points indicate the number of days spent at that altitude and in most instances this was insufficient for acclimatization. (Source: Edwards 1936.) In trying to account for changes in exercise capacity following ascent to high altitude, much of the attention has focused on the various aspects of the oxygen transport cascade described above. In recent years, however, additional consideration has been given to the role of the central and peripheral nervous systems and, in particular, whether hypoxia also affects exercise performance via its impact on these systems. This issue is considered below with some additional details provided in Chapter 12. To investigate these concepts, Amann et al. (2006b) tested the effect of hypoxia (FIO2 = 0.15) and normoxia on peripheral muscle fatigue in eight cyclists. They used magnetic femoral nerve stimulation immediately and 2.5 minutes after constant heavy workloads (314 W) and noted a higher degree of fatigue after the hypoxic ride. Romer et al. (2007) studied nine male cyclists to exhaustion under normoxic and hypoxic conditions (FIO2 = 0.13, SpO2 = 76 ± 1%) conditions and looked at peripheral muscle fatigue with nerve stimulation. Time to exhaustion was reduced by two-thirds under hypoxic conditions, but peripheral fatigue did not differ between the two groups. These findings and those of Katayama et al. (2007) suggest that hypoxia plays a role in exaggerating fatigue and decreasing performance in spite of similar levels of peripheral fatigue. Fatigue may also occur due to reduction in voluntary drive and thus muscle activation (central fatigue). To separate these effects, Amann et al. (2006a) used four repeated time trials of 5 km in trained cyclists with four levels of oxygenation from modest hypoxemic (FIO2 = 0.15) to hyperoxic levels, which resulted in an increase in arterial oxygen content from 17.6 to 24.4 mL O2 dL−l. Peripheral quadriceps fatigue was gauged by a change in force output before and after exercise by magnetic femoral nerve stimulation, and central neural drive (central fatigue) by quadriceps electromyograms. Higher oxygenation resulted in a 43% increase in central nerve output, 30% greater power output and 12% better time trial performance. There was, however, no difference in peripheral muscle fatigue. The authors interpreted their results to mean that there is an effect of hypoxemia on central motor output that reaches a threshold so as not to exceed a critical muscle fatigue. Amann et al. (2007) extended this work and studied eight cyclists to exhaustion under normoxic conditions and two levels of hypoxia (FIO2 = 0.15 and 0.10, SpO2 ∼82% and 67%, respectively). They found that supplemental oxygen prolonged the more severe hypoxic ride, but not the normoxic or moderate hypoxic ride. Moreover, using magnetic
Introduction
Decline in Exercise Performance
Increased dyspnea on exertion
Maximum exercise capacity
Submaximal versus maximal exercise performance
Relevance of the decline in maximum exercise capacity
Mechanism for the decline in maximum exercise capacity
The Lungs
Ventilation
Work of breathing
Work of Breathing at Extremely High Altitudes
Respiratory Pattern
Gas exchange
Ventilation–Perfusion Relationships
Diffusion Limitation
Right-to-Left Shunt
Changes in blood gases
Oxygen-Carrying Capacity
Cardiovascular Responses
Cardiac output
Heart Rate
Cardiac Contractility
Stroke Volume
Pulmonary artery pressure
Locomotor Muscles
Muscle blood flow
Capillary-mitochondria diffusion
Factors Improving Capillary-Mitochondrial Diffusion
Lactate
Central and Peripheral Fatigue and Physical Performance
Stay updated, free articles. Join our Telegram channel
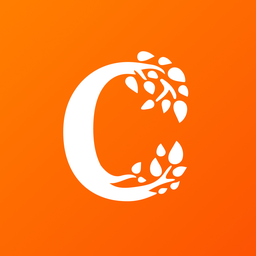
Full access? Get Clinical Tree
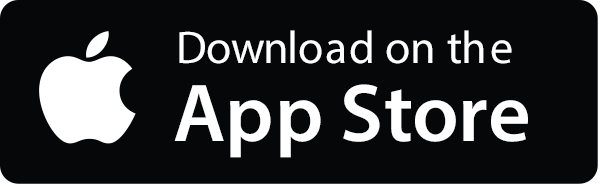
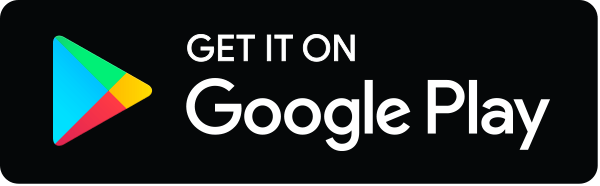