Excitation-Contraction Coupling: Extracellular and Intracellular Calcium Cycles
It is quite … impossible to explain the rapid development of full activity in a [skeletal muscle] twitch by assuming that it is set up by the arrival at any point of some substance diffusing from the surface: diffusion is far too slow. Either we must suppose that [the muscle is stimulated by] excitation (natural or artificial) throughout the interior, not merely at the surface: or we must look for some physical or physico-chemical process which is released by excitation at the surface and then propagated inwards.
—A. V. Hill, 1949
Hill (1949), writing before calcium was discovered to activate the contractile proteins, noted that diffusion of an activator from the surface of a frog sartorius muscle is too slow to account for the rapid onset of the active state in these large, rapidly contracting muscles. This led him to postulate that excitation-contraction coupling, the mechanism by which plasma membrane depolarization initiates contraction, depends on a process more rapid than diffusion across the plasma membrane, or on the release of a diffusible activator from structures within the myocytes. Identification of the role of calcium in activating the contractile proteins (see Chapter 4) led to the discovery that both of Hill’s postulated mechanisms overcome the limitations caused by the slowness of diffusion.
Excitation-contraction coupling in the small, slowly contracting myocytes of primitive and embryonic hearts is effected by an extracellular calcium cycle in which activator calcium diffuses into the cytosol from the extracellular fluid (Fig. 7-1). However, excitation-contraction coupling in the larger myocytes of adult mammalian hearts, which are filled with myofilaments, contract more rapidly, and develop higher levels of tension, requires an additional intracellular calcium cycle. In the latter, depolarization of plasma membrane extensions called transverse tubules (t-tubules) propagates a signal into the cell interior that triggers calcium release from intracellular stores in the sarcoplasmic reticulum (Fig. 7-2) (see Chapter 1).
The Sarcoplasmic Reticulum and Transverse Tubules
The first clue that calcium stored within the sarcoplasmic reticulum activates contraction was obtained in the 1950s, when supernatants obtained after low-speed centrifugation of muscle minces were found to relax actomyosin preparations in vitro (see Chapter 4). This effect, which required the presence of ATP and could be abolished by calcium, was initially believed to be
caused by a “soluble relaxing factor” (Gergely, 1959). However, Hasselbach and Makinose (1961) and Ebashi and Lipmann (1962) discovered independently that the relaxing effect of these supernatant fractions depended on tiny membrane vesicles derived from the sarcoplasmic reticulum, called microsomes, that use energy from ATP hydrolysis to pump calcium into their interior. The concurrent discovery that actin–myosin interactions are activated under physiological conditions by micromolar-ionized calcium concentrations (Weber and Winicur, 1961) made it clear that muscle relaxation is not caused by a soluble factor, but instead occurs when calcium is sequestered by the sarcoplasmic reticulum. Within a few years it was possible to show that the cardiac sarcoplasmic reticulum contains a calcium pump with both sufficient capacity and calcium affinity to relax the heart (Katz and Repke, 1967; Harigaya and Schwartz, 1969).
caused by a “soluble relaxing factor” (Gergely, 1959). However, Hasselbach and Makinose (1961) and Ebashi and Lipmann (1962) discovered independently that the relaxing effect of these supernatant fractions depended on tiny membrane vesicles derived from the sarcoplasmic reticulum, called microsomes, that use energy from ATP hydrolysis to pump calcium into their interior. The concurrent discovery that actin–myosin interactions are activated under physiological conditions by micromolar-ionized calcium concentrations (Weber and Winicur, 1961) made it clear that muscle relaxation is not caused by a soluble factor, but instead occurs when calcium is sequestered by the sarcoplasmic reticulum. Within a few years it was possible to show that the cardiac sarcoplasmic reticulum contains a calcium pump with both sufficient capacity and calcium affinity to relax the heart (Katz and Repke, 1967; Harigaya and Schwartz, 1969).
The answer to the second part of Hill’s question, how a signal generated by activation at the cell surface reaches the interior of the myocytes, was provided when the t-tubules were discovered to be plasma membrane extensions whose lumens open into the extracellular space, and that the t-tubular membranes can propagate action potentials into the interior of the muscle cells. In one of the classic experiments in skeletal muscle physiology, Huxley and Taylor (1958) demonstrated that very small electrical stimuli applied through microelectrodes placed near the mouth of a t-tubule can induce contractions that are limited to the sarcomeres adjacent to the point of stimulation. Further evidence that t-tubules play a critical role in excitation-contraction coupling was obtained when disruption of the connections between the t-tubules and the plasma membrane was found to make it impossible to activate contraction (Eisenberg and Eisenberg, 1968). This and other evidence that transmission of a wave of depolarization down the t-tubules
into the cell interior, which is much more rapid than diffusion of an activator substance, completed the answer to Hill’s question.
into the cell interior, which is much more rapid than diffusion of an activator substance, completed the answer to Hill’s question.
Extracellular and intracellular Calcium Cycles
Muscles can use two possible sources of calcium to activate contraction. As noted above, most of the calcium that activates the small, slowly contracting myocytes of embryonic hearts enters the cytosol from the extracellular fluid (the extracellular calcium cycle), whereas the larger, more rapidly contracting myocytes of the adult mammalian heart depend mainly on calcium derived from intracellular stores (the intracellular calcium cycle). In both cases, the fluxes of activator calcium into the cytosol are passive (downhill) because ionized calcium concentrations in the extracellular fluid and within the sarcoplasmic reticulum are much higher than cytosolic calcium concentration in resting muscle (see Chapter 4).
There are important differences between these calcium cycles. One is that both calcium influx and calcium efflux across the plasma membrane in the extracellular calcium cycle are accompanied by depolarizing currents. In contrast, the calcium fluxes into and out of the sarcoplasmic reticulum in the intracellular calcium cycle do not influence the electrical potential across the plasma membrane. In addition, the electrochemical gradient that drives calcium into the cytosol
across the plasma membrane in the extracellular calcium cycle is increased by the electronegativity within resting cardiac myocytes.
across the plasma membrane in the extracellular calcium cycle is increased by the electronegativity within resting cardiac myocytes.
The relative amounts of calcium delivered to the contractile proteins by the extracellular and intracellular calcium cycles vary among different muscles. In skeletal muscle, virtually all of the calcium that activates contraction is derived from the sarcoplasmic reticulum, whereas most of the activator calcium in smooth muscle and embryonic hearts enters the cytosol from the extracellular space. There are also differences in the sources of activator calcium in the hearts of different mammalian species; in human ventricles, about two-thirds is derived from the sarcoplasmic reticulum and one-third from the extracellular space.
Table 7-1 Structures That Participate in Cardiac Excitation-Contraction Coupling and Relaxation | |||||||||||||||||||||||||||||||||||||||||||||||||||||||||||||||||||||
---|---|---|---|---|---|---|---|---|---|---|---|---|---|---|---|---|---|---|---|---|---|---|---|---|---|---|---|---|---|---|---|---|---|---|---|---|---|---|---|---|---|---|---|---|---|---|---|---|---|---|---|---|---|---|---|---|---|---|---|---|---|---|---|---|---|---|---|---|---|
|
In most mammalian skeletal muscle twitches, enough calcium is released from the sarcoplasmic reticulum to bind to virtually all of the troponin C; as a result, the active state normally reaches its maximum. However, in adult mammalian cardiac myocytes contracting under basal conditions, the sarcoplasmic reticulum provides enough calcium to bind to only ∼40% of the troponin C. This allows the intensity of the contractile response to be modified by variations in calcium release from the sarcoplasmic reticulum, calcium influx across the plasma membrane, and the calcium affinity of troponin C. These variations allow cardiac performance to be regulated by the many structures that regulate calcium fluxes into and out of the cytosol (Table 7-1).
The Extracellular Calcium Cycle
Calcium Influx across the Plasma Membrane
Calcium influx thorough voltage-gated calcium channels participates in both electrical and chemical signaling (Table 7-2). Most important are the L-type calcium channels, named because of their relatively long openings (L = long-lasting); these channels are also called dihydropyridine receptors because they bind with high affinity to this class of calcium channel-blocking drugs. In the atria, ventricles, and His-Purkinje system, where the initial depolarizing current that causes the action potential upstroke is carried by sodium, the subsequent opening of these channels contributes to the action potential plateau by allowing positively charged calcium ions to cross the plasma membrane (see Chapter 14). Analogous depolarizing calcium currents in the SA and AV nodes participate in pacemaker activity and impulse propagation. Calcium entry via L-type calcium channels triggers the release of a larger amount of calcium from intracellular stores in the sarcoplasmic reticulum (see below) and, because some of the calcium that enters the cytosol from the extracellular space is taken up and stored by the sarcoplasmic reticulum where it can be added to the calcium released in subsequent contractions, this calcium influx helps determine the strength of the heartbeat.
Table 7-2 Major Functions of Calcium Entry Through L-Type Calcium Channels in The Heart | ||||||||||||||||||||||||
---|---|---|---|---|---|---|---|---|---|---|---|---|---|---|---|---|---|---|---|---|---|---|---|---|
|
Calcium Efflux across the Plasma Membrane
At any steady state, the calcium that enters the cytosol through L-type calcium channels during each action potential must be pumped out of the cell during diastole. In the heart, two mechanisms effect this uphill transport: a plasma membrane calcium pump and a sodium/calcium exchanger. The latter has a greater capacity than the plasma membrane calcium pump and is responsible for more than 80% of this calcium efflux in human ventricles.
P-Type Ion Pumps: the Plasma Membrane Calcium Pump ATPase (PMCA)
The plasma membrane calcium pump ATPase (PMCA) is one of a family of P-type ion pumps that couple energy derived from ATP hydrolysis to active cation transport; other members of this family include the sarcoplasmic reticulum calcium pump, the sodium pump, and proton pumps (Fig. 7-3). The uphill transport of ions by P-type ion pumps resembles a boat moving upstream through a series of locks (Fig. 7-4). As an ion moves across the membrane bilayer it
becomes “occluded,” which means that it becomes unable to exchange with ions in the aqueous solutions on either side of the membrane—this is analogous to the boat in a closed lock. Transfer of chemical energy from ATP to the occluded ions increases their activity, much as pumping water into the lock raises the boat.
becomes “occluded,” which means that it becomes unable to exchange with ions in the aqueous solutions on either side of the membrane—this is analogous to the boat in a closed lock. Transfer of chemical energy from ATP to the occluded ions increases their activity, much as pumping water into the lock raises the boat.
The turnover of the plasma membrane calcium pump is activated when calcium binds to a transport site on its cytosolic side. Increased cytosolic calcium also stimulates the pump indirectly because in its basal state, when cytosolic calcium is low, the pump is inhibited by a portion of its C-terminal region that lies within the cytosol (Fig. 7-5). Reversal of this inhibition when a calcium-calmodulin complex binds to this C-terminal region of the pump helps cardiac myocytes avoid calcium overload by recognizing increased cytosolic calcium concentration as a signal to accelerate calcium efflux from these cells.
The plasma membrane calcium pump also plays an important role in cell signaling. This occurs when the pump protein is incorporated into caveoli, which allows it to interact with signaling
proteins that include protein kinases, calcineurin, RASSF1 (Ras-associated factor 1), nNOS (neuronal nitric oxide synthase), PDZ domain-containing proteins, and syntrophins that mediate proliferative responses (see Chapters 5 and 9).
proteins that include protein kinases, calcineurin, RASSF1 (Ras-associated factor 1), nNOS (neuronal nitric oxide synthase), PDZ domain-containing proteins, and syntrophins that mediate proliferative responses (see Chapters 5 and 9).
The Sodium/Calcium Exchanger
The sodium/calcium (Na/Ca) exchanger (NCX), the most important mechanism that transports calcium from the cytosol of cardiac myocytes into the extracellular fluid, is an antiport that uses the sodium gradient across the plasma membrane, rather than ATP, to energize the uphill calcium flux. The discovery of this exchanger has a convoluted history. A direct relationship between extracellular calcium and the strength of cardiac contraction was discovered by Ringer in the late 19th century. However, in the 1940s, changes in extracellular calcium were found not to modify the strength of cardiac contraction when sodium concentration was also varied so as to maintain a constant ratio between extracellular calcium and sodium concentrations (Wilbrandt and Koller, 1948). This unexpected observation suggested that calcium and sodium compete for binding to an exchanger that can transport either ion in both directions across the plasma membrane (Láttgau and Niedergerke, 1958). According to this mechanism, binding of calcium to the extracellular side of the exchanger increases calcium influx, which causes the heart to contract more strongly, whereas increasing extracellular sodium weakens contraction by causing the exchanger to bind sodium, rather than calcium, for transport into the cell. Evidence was also obtained that calcium efflux is determined by the relative concentrations of sodium and calcium at the intracellular side of the exchanger. The importance of Na/Ca exchange was established by Reuter and Seitz (1968), who found that the exchanger accounts for ∼80% of the calcium efflux from the mammalian myocardium.
A simple way to understand Na/Ca exchange is to view the exchanger as a carrier that, after binding either sodium or calcium both within and outside the cell, shuttles these ions in opposite directions across the lipid bilayer (Fig. 7-6). The exchange is reversible, its direction depending on the electrochemical activities of sodium and calcium on either side of the membrane. Although the driving force for uphill calcium transport out of the cytosol is the sodium gradient across the plasma membrane, the ultimate source of this energy is the ATP used by the sodium pump to establish the sodium gradient.
NCXs are intrinsic membrane proteins that include 10 membrane-spanning α-helices and a large cytosolic domain (Fig. 7-7). One α-helix is a signal peptide that is removed from the functional exchanger in some members of this family. The remaining nine α-helices, along with the intervening amino acid sequences, are organized in two hydrophobic clusters of five and four α-helices, which together participate in cation exchange across the plasma membrane. The intracellular domain contains sites that modify the activity of the exchanger in response to protein kinase-catalyzed phosphorylations, changes in cytosolic calcium, and an allosteric effect of ATP.
Although Na/Ca exchange does not require ATP hydrolysis, its turnover is stimulated by high concentrations of ATP. This allosteric effect is one example of the general ability of ATP to accelerate ion fluxes that are mediated by exchangers, channels, and pumps. A corollary to this effect is that Na/Ca exchange is inhibited when ATP concentration falls which, by reducing calcium efflux, can exacerbate calcium overload in energy-starved hearts. The exchanger is activated when it is phosphorylated by protein kinases A and C, and by calcium-calmodulin-dependent protein kinase (CAM kinase). In addition, elevated intracellular calcium increases the turnover of the exchanger by a regulatory effect that, like phosphorylation by CAM kinase, helps alleviate calcium overload.
The NCX is electrogenic because it exchanges three sodium ions in exchange for one calcium ion; a ratio of 4:1 has also been described (Dong et al., 2002). This generates an ionic current, defined as the flux of positive charge, that flows in the same direction as the sodium flux and is opposite to the movement of calcium (Fig. 7-6) (this can be remembered as “current follows sodium”). The currents generated by the exchanger are normally small, and contribute only a few millivolts to membrane potential. However, they can cause dangerous arrhythmias in patients with calcium-overloaded hearts, where calcium efflux across the plasma membrane is increased (see Chapters 14, 16, 17, and 18).
The electrogenicity of Na/Ca exchange also allows membrane potential to influence the direction of ion transport by the exchanger. In the resting heart, the negative intracellular potential favors the entry of positively charged sodium ions, and so promotes calcium efflux. Reversal of membrane potential during systole, when the inside of the cell becomes positively charged, has the opposite effect, to increase calcium influx by favoring sodium efflux. These effects of membrane potential help the NCX operate in a manner that favors calcium flux in whatever direction maintains the existing mechanical state: calcium efflux—which relaxes the heart—is favored by the intracellular negativity during diastole, and calcium influx—which increases contractility—is favored during systole.
Discovery of Na/Ca exchange provided the key to understanding the positive inotropic effect of cardiac glycosides, which in the 1950s had been discovered to inhibit sodium transport out of cells (Schátzmann, 1953). These drugs increase myocardial contractility because the increased intracellular sodium caused by sodium pump inhibition favors sodium efflux by the exchanger, which reduces calcium efflux. By retaining calcium within the myocytes, this response increases contractility by adding to the amount of calcium available for release during excitation-contraction coupling (Repke, 1964).
Other Ion Fluxes across the Plasma Membrane
The Sodium Pump
The sodium pump (also called the sodium-potassium ATPase or Na-K-ATPase because its ability to hydrolyze ATP is stimulated when sodium and potassium are present together) transports sodium out of the cytosol in exchange for potassium that enters the cell. This exchange of sodium and potassium cations reduces the electrochemical work of the sodium pump. However, both sodium efflux and potassium influx are uphill processes, so that the sodium pump requires energy that is derived from ATP hydrolysis. The impact of the sodium pump on cellular energy expenditure is high, as evidenced by the fact that the sodium pump accounts for 20% to 30% of the ATP consumed by non-motile tissues like the kidneys.
The major function of the sodium pump in working cardiac myocytes is to exchange the small amount of sodium that enters the cytosol during each action potential with some of the potassium that leaves the cytosol during repolarization. The electrochemical gradients established by the sodium pump also contribute to the driving force for the sodium currents that depolarize the working myocytes of the atria and ventricles, and the Purkinje fibers, and for the potassium currents that repolarize all regions of the heart (Chapter 13). The sodium gradient across the plasma membrane energizes calcium efflux by the NCX (see above), proton efflux by the NHE (see below), and the active transport of several substrates and metabolites across the plasma membrane.
The sodium pump includes three subunits (Fig. 7-8). The largest is the α-subunit, a P-type ion pump ATPase (see Fig. 7-3) that contains 10 membrane-spanning α-helices and a large intracellular domain. The sites that bind potassium for transport into the cytosol are on the extracellular side of the α-subunit, while sodium binds on the cytosolic side. The smaller β-subunit, which is a glycoprotein that includes a single membrane-spanning domain, participates in trafficking of the sodium pump to the plasma membrane and regulates cation-binding affinity. The γ-subunit (phospholemman) is a substrate for phosphorylations by protein kinases A and C that stimulate the sodium pump. Pump activity is also regulated by acylation of the α-subunit, glycosylation of the β-subunit, and an allosteric effect of high
ATP concentration that stimulates the pump. Long-term changes in pump activity, seen in diseased hearts, result from isoform shifts and changes in the concentration of the pump in the plasma membrane (see chapter 18).
ATP concentration that stimulates the pump. Long-term changes in pump activity, seen in diseased hearts, result from isoform shifts and changes in the concentration of the pump in the plasma membrane (see chapter 18).
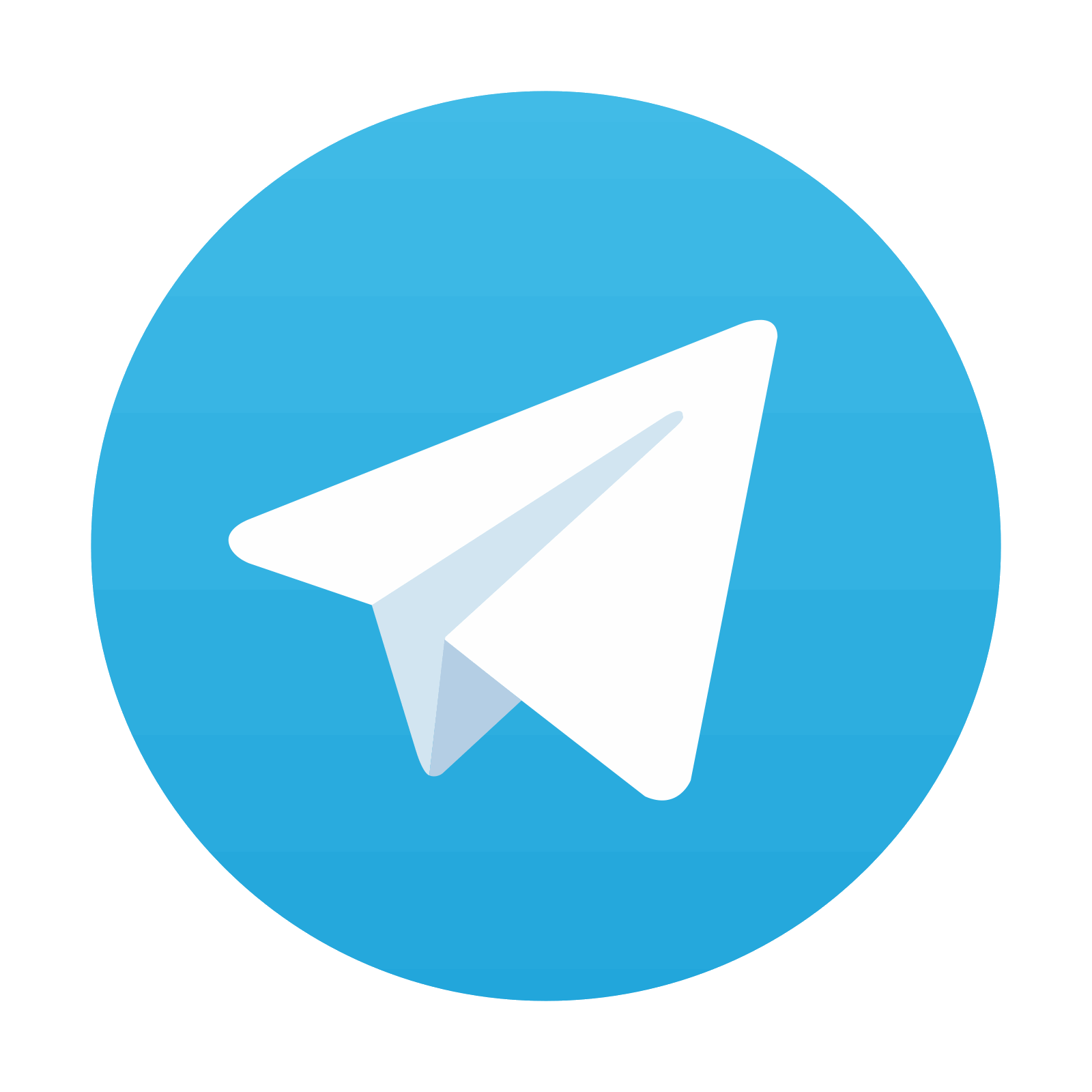
Stay updated, free articles. Join our Telegram channel
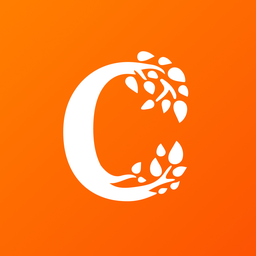
Full access? Get Clinical Tree
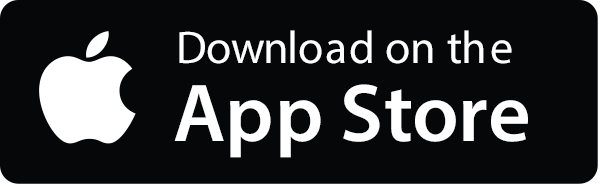
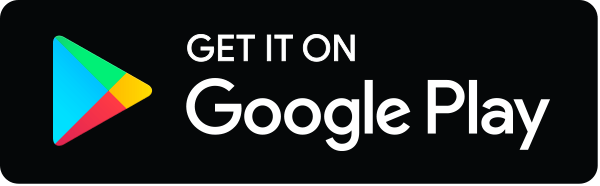
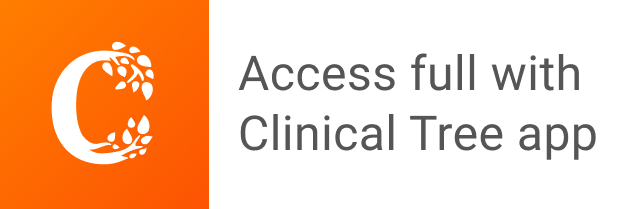