INTRODUCTION
Recent advances in image acquisition techniques and scanner hardware have allowed magnetic resonance imaging (MRI) to become a useful tool in the noninvasive assessment of cardiovascular diseases. In addition to high-resolution anatomical images, cardiovascular magnetic resonance (CMR) provides quantitative assessment of ventricular function, myocardial perfusion, viability, and shunt flow and measurements of valvular velocities and gradients. In addition, coronary, pulmonary, and systemic vasculatures can be assessed with contrast-enhanced magnetic resonance angiography (MRA) without the use of ionizing radiation or nephrotoxic contrast agents. CMR’s comprehensive ability to image the heart also makes it a useful tool in the evaluation of diastolic function.
PRINCIPLES OF MAGNETIC RESONANCE IMAGING
Physics of Magnetic Resonance Imaging
All atoms have nuclei that are composed of one or more protons. These protons have a small positive electric charge and spin at a rapid rate. The rapid spinning motion of a positively charged proton produces a very small but measurable magnetic field that in a sense is like a tiny bar magnet. Normally, the magnetic fields of these protons are randomly oriented throughout the body. When placed within an MRI scanner, the protons within the body will align themselves with the external magnetic field of the scanner, just like a compass would align itself with Earth’s magnetic field. By applying specific radiofrequency (RF) waves, some of these protons will change their alignment (“resonate”) to a more excited state. As these protons relax and return to their original alignment, they give off an RF signal that can be measured and used to generate a clinical image. Since hydrogen ( 1 H) is the most abundant atom in the body capable of generating a clinically useful image, 1 H protons form the basis of clinical MRI.
Basic Imaging Sequences
MRI depends on using gradient coils within the scanner to send RF pulses in specific patterns to stimulate the 1 H protons within the body. As these protons relax, they emit signals that are detected by receiver coils placed over the surface of the body. Pulse sequences are combinations of different types of RF pulses that are placed together to create images with specific characteristics. Combinations of different pulse sequences can be used to evaluate parameters that define both global and regional diastolic functions. These parameters include: flow mapping of the mitral valve, pulmonary vein, and tricuspid valve; global and regional rotation; translation and radial motion; peak filling rate (PFR) and time-to-peak filling rate (TPFR) of contrast; and phosphorus-31 magnetic resonance ( 31 P-MR) spectroscopy to measure myocardial energy content. The most common types of pulse sequences relevant to diastolic function are the following.
Spin Echo or “Black Blood” Images
These pulse sequences are designed so that flowing blood produces no signal and appears black ( Fig. 8-1A ). However, because of the time required to stimulate and then saturate the signal emitted from the flowing blood, spin echo pulse sequences produce still images. Spin echo sequences can be “weighted” to highlight different relaxation properties of the protons, resulting in T1 (T1w) or T2 (T2w) weighted images. Although spin echo sequences are not used specifically to evaluate cardiac diastolic function, they do provide good tissue contrast and anatomical detail, making them useful for visualizing morphology.

Gradient Echo or “White Blood” Images
These pulse sequences are fast enough to “catch” the signal coming from excited blood so that blood appears white ( Fig. 8-1B ). Gradient echo (GRE) sequences produce cine images with good temporal resolution (typically 20–30 fps; up to 70 fps for perfusion imaging). GRE sequences are used for evaluating cardiac function as well as turbulent flow due to valvular disease or intracardiac shunts.
GRE sequences yield accurate and highly reproducible measurements of ventricular volumes and indices of cardiac systolic function without the need for geometric assumptions. From the contours describing the endocardial and epicardial borders of the myocardium, time-volume curves can be derived that assess global diastolic function ( Fig. 8-2 ). The peak filling rate (PFR) from these curves describes the maximum change in cm 2 per second during the rapid filling phase of ventricular systole, whereas time to PFR is measured between end systole and the point at which PFR occurs. Both PFR and time to PFR are typically prolonged when diastolic function is impaired.

Phase Velocity or Phase Contrast Imaging
As hydrogen nuclei move through a magnetic field, they shift their particular phase (the manner in which protons rotate about a particular axis) in a way that is proportional to their velocity. Phase-contrast MRI (PC-MRI), which is somewhat analogous to pulsed-wave Doppler echocardiography, takes advantage of this change in phase with velocity to measure the velocity of blood moving through an area of interest. Clinically, PC-MRI is used to calculate transvalvular velocities as well as gradients, regurgitant volumes, and ratios of pulmonary-to-systemic blood flows (Qp/Qs). Flow mapping of mitral and tricuspid valve inflow using PC-MRI allows accurate assessment of peak velocities and volume flow of early (E) and late or atrial (A) filling waves ( Fig. 8-3 ), showing good correlation with Doppler-derived data. Similar techniques are also used to map pulmonary and caval venous flow ( Fig. 8-4 ). More recently, PC-MRI measurements of tissue velocities have been used to quantify myocardial velocities, enabling calculation of local strain. Strain rate can also be quantified for regions of myocardium as well as for each myocardial voxel.


Myocardial Tagging
A unique feature of CMR is that it allows the myocardium to be magnetically labeled with a rectangular or radial grid that acts as a marker of myocardial deformation during contraction ( Fig. 8-5 ) and also shows abnormal myocardial tagging. The creation of tags requires the application of specific RF pre-pulses in one or more planes perpendicular to the imaging plane, prior to the application of RF pulses that are used for cine (bright blood) imaging. The tagging pre-pulses cause the tissue in the tag planes to be “saturated” so that they appear as hypointense or black lines compared with the normal surrounding tissue. By measuring tag displacement and deformation during the cardiac cycle, myocardial deformation can be analyzed, which serves as the basis for strain and strain rate analysis using CMR ( Fig. 8-6 ). Images can be obtained every 20 msec, allowing very high temporal resolution. Clinically, myocardial tagging is used in the evaluation of diastolic function, of abnormal myocardial deformation in patients with hypertrophic cardiomyopathy (HCM) or complex congenital heart disease, and of pericardial motion in patients with suspected constrictive pericarditis and in the differentiation of solid structures (e.g., muscular tissue from tumor or thrombus). Different myocardial tagging techniques are currently available that allow evaluation of myocardial deformation in both two-dimensions and three-dimensions.


Delayed Hyperenhancement
In areas where the myocardium is scarred or fibrotic, there is delayed “wash-in” and “wash-out” of MRI contrast agents. These fibrotic or scarred areas show up as bright or “hyperenhanced” areas of myocardium when “delayed” images are taken, typically 10–15 minutes after injection of gadolinium-DTPA (diethylenetriamine penta-acetic acid) (see Fig. 8-1C ). A special inversion signal given prior to the main pulse sequence is used to “null” the signal from the normal myocardium so that it can be more easily distinguished from abnormal, hyperenhanced myocardium. Specific patterns of hyperenhancement correspond with certain cardiovascular diseases and can be used to distinguish ischemic from non-ischemic cardiomyopathy, as well as to differentiate among different forms of infiltrative cardiomyopathies ( Fig. 8-7 ).

Magnetic Resonance Spectroscopy
Besides 1 H, other magnetic nuclei in the human body, such as 23 Na, 13 C, 19 F, and 31 P, can also generate MRI signals, though normally with much less intensity and under much more technically demanding conditions. 31 P-MR spectroscopy provides a profile of regional adenosine triphosphate (ATP) and phosphocreatine (PCr) contents and thus an estimate of energy status and viability of the myocardium. Myocardial relaxation is an active, energy-dependent process, and changes in the ratio of myocardial PCr and ATP levels (PCr/ATP) by 31 P-MR spectroscopy are seen in abnormal diastolic function. On a cellular level, these abnormalities in PCr/ATP ratios are thought to result in impaired Ca 2+ sequestration, leading to impaired relaxation in cardiac myocytes.
CLINICAL RELEVANCE
This section reviews the role of CMR in evaluating diastolic function in a spectrum of cardiac disorders, including HCM, ischemic heart disease, constrictive pericarditis, and restrictive cardiomyopathies.
Hypertrophic Cardiomyopathy
The characteristic finding of HCM is an inappropriate myocardial hypertrophy in the absence of an obvious cause, such as aortic stenosis or hypertension. Although transthoracic echocardiography is the primary imaging modality in HCM, CMR is an excellent alternative in the diagnosis and follow-up of patients. The array of MRI sequences used in the evaluation of HCM includes spin echo MRI, GRE MRI, PC-MRI, MRI tagging, delayed enhancement (DE), and MR spectroscopy. A combination of these sequences is used to identify the presence and severity of hypertrophied myocardium, to distinguish different forms of HCM, to differentiate obstructive from nonobstructive forms of the disease, and to define prognosis.
Diastolic dysfunction is thought to be one of the major pathophysiological mechanisms in patients with HCM, frequently leading to diastolic heart failure. These diagnostic abnormalities can be evaluated using cine tagging, DE, PC-MRI, and MRI spectroscopy sequences.
The effect of myocardial hypertrophy on regional and global left ventricular (LV) and right ventricular (RV) function can be precisely characterized by MRI. Global systolic function in HCM is often increased with high ejection fractions and low end systolic volumes. In the hypertrophied myocardium, however, cine tagging sequences demonstrate reduced or absent systolic wall thickening, which is related to muscle disorganization ( Fig. 8-8 ). Strain analysis in these same regions show that systolic myocardial strains are invariably decreased, with reduced longitudinal and circumferential shortening. Three-dimensional CMR tagging demonstrates specific patterns of myocardial deformation among the different forms of HCM, although all forms have abnormally small circumferential curvatures in hypertrophied segments.

Abnormal DE of the myocardium after imaging by gadolinium-DTPA is found in approximately 80% of patients with HCM. Invariably, the enhancement occurs in the hypertrophied regions, with a mean volume of enhancement of 8% to 11% of LV mass. The pattern of enhancement is usually patchy with multiple foci, predominantly involving the middle third of the ventricular wall, especially near the insertion points of the RV free walls onto the interventricular septum (see Fig. 8-1C ). This pattern of enhancement is distinct from the predominantly subendocardial distribution of hyperenhancement seen in patients with coronary artery disease. Histologically, these abnormally enhanced areas of myocardium are thought to represent scarred myocardium due to microinfarction or are in relation to myocardial disarray and consequent interstitial expansion. In a highly select group of patients with HCM, the degree of myocardial enhancement was associated with progressive disease and increased risk for sudden cardiac death. It has been proposed that the degree of diastolic dysfunction is linked to the extent of myocardial fibrosis quantified by DE-MRI.
Impaired myocardial relaxation can be identified using GRE-derived time-volume curves of the left ventricle, which demonstrate prolonged time-to-peak rapid filling and time-to-peak wall thickness thinning rates. In 31 patients with HCM, regional prolongation of time-to-peak wall thinning rate was detected in segments with extensive myocardial fibrosis and decreased perfusion. Other MRI techniques, such as 31 P-MR spectroscopy, demonstrate abnormal myocardial high-energy phosphate metabolites in both symptomatic and asymptomatic patients with HCM. Lower myocardial energy content is thought to contribute to relaxation abnormalities in hypertrophied hearts. Finally, PC-MRI of flow patterns in the caval and pulmonary veins, as well as the diastolic inflow in the tricuspid/mitral valves, can be used to evaluate diastolic function, similar to echocardiography.
Hypertension and Aortic Stenosis
Chronic pressure overload in systemic hypertension and aortic stenosis leads to hypertrophy of the left ventricle, with the addition of new sarcomeres in parallel to existing ones to normalize wall tension. Hypertrophy, coupled with a variable degree of altered passive elastic properties of the myocardium, leads to diastolic dysfunction. In LV hypertrophy, changes in ventricular time-volume curves, including prolonged TPFR, early-to-late filling ratio, and early filling percentage measured from GRE sequences, can be demonstrated before changes in the classical mitral Doppler pattern. Other early changes include a decreased PCr/ATP ratio on 31 P-MR spectroscopy, even before the development of evident LV hypertrophy. Later in hypertrophy, PC-MRI demonstrates typical changes in mitral valve inflow that are similar to the classical mitral Doppler patterns. CMR-tagged data demonstrate a delay and prolongation of diastolic untwisting, as well as a reduction in regional strain in patients with LV hypertrophy in comparison with normals.
Coronary Artery Disease
Resting impairment of diastolic function is common in ischemic heart disease. Such diastolic dysfunction precedes systolic dysfunction and occurs as the earliest sign of myocardial ischemia. Diastolic filling patterns in ischemic heart disease are often complex, reflecting regional heterogeneity of myocardial function due to different degrees of ischemia, infarction, postinfarction remodeling, and myocardial stunning and hibernation.
Three-dimensional tagging by CMR is well suited to assess these regional changes in diastolic function. In a model of post-infarction LV remodeling, a reduction of regional strains was observed in both the infarcted region and, to a lesser extent, the remote regions. Diastolic untwisting tended to be nonuniform, delayed, and prolonged in areas of both infarcted and hibernating myocardium. Similar changes were seen in an animal model of transmural ischemia, with restoration of the normal untwisting motion after reperfusion. PC-MRI can be used to evaluate regional diastolic function by quantifying tissue velocities in patients with previous myocardial infarction. PC-MRI measurements of mitral valve inflow after myocardial infarction demonstrate the typical changes in transvalvular flow patterns and closely correlate with Doppler echocardiography.
Dilated Cardiomyopathy
While the diagnosis of dilated cardiomyopathy can be easily made with transthoracic echocardiography, CMR can provide a comprehensive evaluation of cardiac function, myocardial stress perfusion, and viability. CMR has been shown to reliably discriminate between ischemic and non-ischemic causes of cardiomyopathy, and CMR remains the gold standard for the evaluation of LV and RV volumes, masses, ejection fractions, and viabilities.
Diastolic dysfunction is common in dilated cardiomyopathy, and its severity is related to prognosis. The same CMR techniques previously described can be used for the comprehensive evaluation of both systolic and diastolic function in these patients’GRE-based time-volume curves for analysis of global diastolic function; PC-MRI to determine inflow curves as well as tissue velocities; myocardial tagging to evaluate strain and, more recently, ventricular dyssynchrony; and 31 P-MR spectroscopy to determine PCr/ATP ratios as a measure of myocardial energy content.
Constrictive Pericarditis
The pericardium is well characterized by CMR because of its excellent contrast resolution and multiplanar imaging capability. Typically, T1- and T2-weighted spin echo, GRE, and tagged cine sequences are used in patients with suspected pericardial disease to evaluate cardiac function and morphology, pericardial thickness and tethering, and the presence of ventricular interdependence. In patients with acute pericarditis, T2-weighted spin echo or DE images after administration of gadolinium-DTPA can be used to detect pericardial inflammation and edema.
On T1-weighted spin echo images, the normal pericardium is most easily identified over the right ventricle and appears as a thin band of low signal intensity between the high signal intensities of the external pericardial fat and the internal epicardial fat ( Fig. 8-9 ). The low signal intensity of the pericardium on CMR images is attributable to the fibrous, low proton density component of the pericardium in combination with a small quantity of pericardial fluid. The normal pericardial thickness is approximately 2 mm, although thicknesses of up to 4 mm are not necessarily abnormal. The appearance of pericardial effusions may vary depending on the protein and cellular content of the effusion; transudative pericardial effusions appear dark on T1-weighted spin echo images and bright on T2-weighted images due to their high water content. Exudative effusions, which have relatively lower water content, have the opposite appearance. In patients with pericardial effusions, GRE sequences are useful to distinguish the pericardium from pericardial fluid. The latter typically has a bright appearance on GRE sequences.
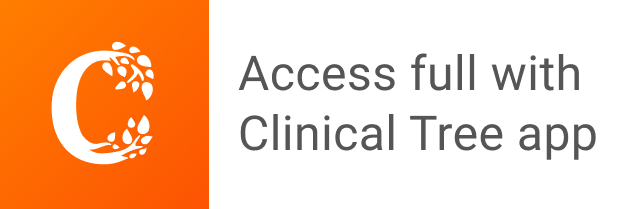