Magnitude of weight loss at high altitude Body composition during weight loss at high altitude Energy deficit at high altitude Mechanisms for the energy deficit and weight loss Nutrition and metabolism in high altitude residents Water balance at high altitude Weight loss is a very common phenomenon associated with travel to high altitude, particularly with long exposures to the extremes of elevation. The mechanisms for this phenomenon, which is nearly universal with travel above 5000 m, vary significantly in magnitude between individuals and have remained unclear. Anorexia and decreased energy intake have long been suspected of playing a large role and may, in turn, be related to changes in neuroendocrine function and subsequent effects on taste, hunger, and satiety, as well as altitude illness and alterations in gastrointestinal function or protein metabolism. The goal of this chapter is to explore these issues in greater detail. This chapter begins by describing the extent of weight loss at high altitude and the consequences for body composition. It then reviews the changes in energy balance following ascent and considers the mechanisms responsible for these changes and the observed weight loss. After providing an overview of strategies for mitigating weight loss through alterations in dietary intake, the chapter considers alterations in energy balance and metabolism in long-term residence as well as the influence of high altitude on water balance. It is generally well established that chronic exposure to high altitude is associated with a decrease in body mass as a result of a chronic energy imbalance or deficiency. The results of a recent meta-analysis on the topic of weight loss at high altitude (Dünnwald et al. 2019) are illustrated in Figure 15.1 and reveal a progressive decrease in mean body weight, fat-free mass, and fat mass in proportion to the subgroups of high altitude exposure: moderate (1500–3500 m), high (3500–5300 m), and extreme altitude (>5300 m). Changes reported in each of these altitude ranges are described below. Figure 15.1Forest plot from random-effects meta-analysis of mean body weight changes (95% confidence intervals) in the subgroups of moderate (1500–3500 m), high (3500–5300 m), and extreme (>5300 m) altitude. As noted in the overall averages, there is a progressive increase in average weight loss from moderate (–1.7 kg) to extreme (–4.9 kg) altitude. Plots B and C denote the corresponding losses (mean [95% CI]), where available, in fat-free mass and fat mass. Data redrawn and modified from previous work (Dünnwald et al. 2019) and comprise >30 published studies. For full references, see: Dünnwald et al. (2019). Most members of climbing and trekking groups experience weight loss in the initial first three weeks of an expedition, even when walking below 3000 m. This is probably due to the change in lifestyle for most subjects from an urban semisedentary existence to the more active lifestyle of walking four to eight hours a day with some considerable ascents and descents. In addition to reduced food availability and frequency of meals while trekking, gastrointestinal infections, including traveler’s diarrhea and gastroenteritis, are common. Boyer and Blume (1984) found that 13 members of the American Medical Research Expedition to Everest (AMREE), during the trek into the Everest region (i.e., from 2800 to 5300 m), lost an average of 2 kg (range 0–6 kg). Those with the highest percentage of body fat to start were reported to lose the most weight. Two subjects with less than 13% of body fat lost no weight. Dinmore et al. (1994) similarly found an average loss of 1.3 kg during the first week of trekking but only a further 0.5 kg in the next week. These early studies are consistent with a meta-analysis (Dünnwald et al. 2019) of reductions in mean body weight of 1.7 kg as illustrated in Figure 15.1a. Although some studies have demonstrated that it is possible to attenuate high altitude induced weight loss by increasing caloric intake (Butterfield et al. 1992; Rai et al. 1975), the bulk of studies to date demonstrate that modest weight loss (2.3 kg; Dünnwald et al. 2019) is common during the typical trekking profiles (Figure 15.1a). After partial acclimatization, weight loss is usually seen with travel to elevations above 5300 m with an average loss of about 4.9 kg (Dünnwald et al. 2019) (Figure 15.1a). Figure 15.2 shows the extreme effect of altitude on body weight on one well-acclimatized subject during the 1960–61 Silver Hut expedition. Following an initial decline of 5.3 kg during the trek into the location of the hut, the individual steadily lost weight at a weekly rate of just under 400 g week−1 but, on two occasions, on descent to altitudes of 4000–4500 m, began to gain weight. Most subjects in the Silver Hut expedition lost between 0.5 and 1.5 kg week−1 (Pugh 1962). Figure 15.2Record of body weight of one subject during the Silver Hut Expedition 1960–61. After the march out from Kathmandu (K) and the initial period of preparation, he was in residence at 5800 m (hatched areas) or at base camp at 4500 m. Note the loss of weight at 5800 m but weight gain during two breaks at 4500 m. These early observations from the Silver Hut expedition are broadly consistent with Dinmore et al. (1994) who observed an average weight loss of 3.9 kg during two weeks of climbing above 5000 m. On the 1992 British Winter Everest Expedition, a mean weight loss of 5 kg was observed above 5400 m out of a total loss of 7.8 kg for the entire expedition (Travis et al. 1993). Exposure to cold temperatures is an unavoidable aspect of most high altitude expeditions; however, the execution of an Everest expedition during winter can only have compounded the effects of cold exposure on body weight and composition. At advanced base camp (6300 m) during AMREE, most subjects lost weight. Boyer and Blume (1984) documented an average loss of 4 kg (range 0–8 kg) over a mean of 47 days in 13 subjects, with considerable interindividual variation based on the initial percentage of body fat. Interestingly, Boyer and Blume also found that Sherpas, who averaged only half as much body fat as the Western climbers, lost no weight during the time spent above base camp, mostly at or above 6300 m. A possible reason for differences in weight loss in response to high altitude is the athletic fitness of subjects and the extent of fat mass at sea level. It is well known that the Sherpas and Nepali porters are well accustomed to long-term activities with high energy expenditure (and also have very low fat mass) and, as such, have likely evolved mechanisms to maintain appropriate energy balance. There is some evidence that females may lose less weight than males at high altitude. Hannon et al. (1976) found their female subjects lost an average of only 1.8% of body weight during seven days at 4300 m, whereas previously reported data from males at this altitude found losses of 3.5–5.0% (Fulco et al. 1985). Collier et al. (1997) observed changes in body mass index at Everest base camp (5340 m) over a median of 15 days: 22 males lost 110 g m−2 day−1 compared with 20 g m−2 day−1 in eight females, a significant difference (p = 0.03). The seven male climbers who climbed to between 7100 m and 8848 m, using oxygen at extreme altitude, all lost weight, averaging 150 g m−2 day−1. The one female climber who spent four nights above 8000 m without supplementary oxygen lost no weight between leaving and arriving back at base camp. These initial studies and observations needed to be confirmed in larger sample sizes. The mechanism(s) explaining this stability of body mass in females were not explained but likely involve less muscle and/or fat mass, lower total daily energy expenditure (TDEE) and/or basal metabolic rate (BMR), and some protective influences of female sex hormones. It could be argued that some of the weight loss on expeditions is due to cold, limited food supplies, and the increased energy expenditure of trekking and/or climbing. Chamber studies avoid this potential criticism and provide a means to isolate the effects of hypoxia per se from these other aforementioned factors. Although most chamber studies are of too short a duration to be relevant, Operation Everest I and II, studies of 40 days’ duration each, showed that despite stable environmental conditions of temperature and humidity, and diet ad libitum, subjects still lost weight (Rose et al. 1988). In Operation Everest II, six subjects lost an average of 7.4 kg during the 38 days of observations as they ascended the simulated height of the summit of Everest. Energy intake fell by 43% and, interestingly, the subjects chose a diet that resulted in a reduction of carbohydrate from 62% to 53% of the total diet. The authors considered that the weight loss could not be accounted for solely by the reduction in intake and that malabsorption of nutrients or increase in energy expenditure due to increased BMR must be invoked. The exercise taken in this chamber study would probably be much less than on a climbing expedition; and while exercise equipment was available, only 20% of estimated basal energy expenditure was added to the subject’s routine activity, which would seem to have unlikely compensated for the confinement-induced reductions in normal daily activity. On Operation Everest III (Comex ’97) there was a similar loss of weight, averaging 5 kg during the 31-day chamber study taking eight subjects to the simulated height of the Everest summit. Intake was reduced by 4.2 MJ day−1 due to subjects feeling satiated sooner (Westerterp-Plantenga et al. 1999). Assuming much of the weight loss is due to negative energy balance (see the “Energy Deficit at High Altitude” section later in this chapter), a simplistic view is that the body would use up fat stores along with skeletal muscle proteins. More realistically, however, the body uses concurrent stores of fat, glycogen, and, to a lesser extent, muscle mass, with the contribution from each varying based on the intensity of the activity (i.e., fat during rest to mostly glycogen during higher intensity exercise) and the level of protein intake, which helps to preserve muscle mass. Although this does not seem to be the case at high altitude as detailed in later sections, several studies at sea level using high protein intake with or without resistance training have been able to limit or even preserve muscle mass reduction during weight loss (reviewed in: Cava et al. 2017; Liao et al. 2017). The results of a recent meta-analysis on the topic of body composition at high altitude (Dünnwald et al. 2019) are illustrated in Figure 15.1 and show clear reductions in body mass, including both fat-free mass (FFM; Figure 15.1b) and fat mass (FM; Figure 15.1c), with larger changes occurring at higher altitudes. At high altitude, diet (21%) and expedition duration (20%) explain most of the heterogeneity in these changes in body composition between studies (Dünnwald et al. 2019). Physiological sex and diet explain 66% and 42% of the between-study heterogeneity in FFM changes, respectively, whereas diet and type of exposure (active [trekking/climbing, etc]/passive [simulated altitude or low level activity]) explain 25% and 30% of between-study heterogeneity in changes in FM, respectively. At high altitude, as at moderate altitude, the pooled mean decreases in body weight were higher with active exposure and when diet was not manipulated or when the diet was hypocaloric. However, even when the caloric intake is matched or increased relative to energy output, body weight is still significantly decreased by –2.5 to –0.4 kg, indicating hypoxia to be a possible trigger for body composition changes in these studies (Barnholt et al. 2006; Bharadwaj and Malhotra 1974; Butterfield et al. 1992; Consolazio et al. 1972; Holm et al. 2010; Surks et al. 1966). Perhaps not surprisingly, studies that revealed the highest mean reductions in body weight at high altitude were those associated with more prolonged durations of exposure. In these studies, on average, FFM and FM affected the pooled mean body weight changes to a similar extent. At extreme altitude, as proposed earlier, baseline body weight accounts for most of the heterogeneity in body weight changes between studies (84%), whereas the duration of exposure accounts for 14%. In the Operation Everest II study (Rose et al. 1988), there was a loss of 2.5 kg of FM (1.6% body weight) and 4.9 kg of FFM. Computed tomographic examination of the thigh showed a 17% loss of muscle and a 34% loss of subcutaneous fat. These results of reductions in both FM and FFM were confirmed in a very recent study that showed that there is muscle wasting even during short simulated exposures (21 days) to an altitude of only 4000 m under strictly controlled and standardized environmental, dietary, and activity conditions (Debevec et al. 2018). An important driver of weight loss and changes in body composition seen with travel to very high elevation is the emergence of an energy deficit. The widely accepted view is that energy balance in humans is determined via the interplay between energy intake and energy expenditure. However, this view is limited because it considers energy intake and expenditure to be independent parameters that can be adjusted at will and thereafter remain static without being influenced by homeostatic signals related to weight loss (Hall and Chow 2013). It is more appropriate to conceptualize energy intake and expenditure as interdependent variables that are dynamically influenced by each other and body weight (Hall et al. 2012). Ascent to, and life at, high altitude provide examples of such complex challenges to energy balance. While recognizing the complexities of energy balance, it is useful to define the main components of energy expenditure and energy intake. Total daily energy expenditure (TDEE) consists of three components: (1) expenditure for maintenance processes, usually called resting energy expenditure or basal metabolic rate (BMR); (2) thermogenesis from the processing of ingested food, referred to as diet-induced thermogenesis; and (3) energy cost of physical activity or activity-induced energy expenditure (AEE). Energy intake is influenced by appetite, including the palatability of food, gastrointestinal function, and the availability of food. The main determinants of energy balance are illustrated in Figure 15.3, while the alterations and modifying factors at high altitude are outlined in the following sections. Figure 15.3Potential factors involved in the regulation of energy balance during ascent and stay at high altitude EE, energy expenditure.. FFM, fat-free mass. FM, fat mass. MM, muscle mass. BW, body weight. Nair et al. (1971) found that after a week at 3300 m, the BMR was elevated by about 12% versus sea level. Exposure to cold and hypoxia in a second group of subjects made no difference in this effect compared with hypoxia alone. BMR returned to sea level values by week 2 and even fell below these values by week 3. With additional cold exposure in these hypoxic conditions, BMR rose above sea level values by week five and remained elevated a week after return to sea level. In seven healthy males, Butterfield et al. (1992) found BMR to be elevated by 27% on day 2 at Pikes Peak (4300 m) in Colorado; this increase in BMR was attenuated to 17% above baseline on day 10 and remained stable at this level for the next 11 days. In 16 females, also studied at 4300 m and while controlling for menstrual phase, it was found that BMR was elevated by 7% after three days but had returned to sea-level values by day six (Mawson et al. 2000). Although it was speculated that the high levels of fitness of the subjects could explain the temporal changes in BMR, the energy requirements to assure weight maintenance and composition remained 6% elevated above sea-level values giving rise to an apparent “energy requirement excess” of about 670 kJ/day; therefore, it is possible that this excess in energy could also influence the changes in BMR. After 82 to 113 days of acclimatization, BMR measured at 5800 m was elevated, on average, by about 10% (Gill and Pugh 1964). It is likely that BMR rises even more if subjects climb to very high altitudes without acclimatization. Although there are currently no data on BMR at altitudes above 6000 m, it is plausible that ascent to such elevations is a factor in the more pronounced weight loss that has been reported. Sympathetic activity is increased at high altitude (Chapter 16) and the finding that ß-blocker administration blunts the increase in metabolic rate (Moore et al. 1987) suggests it is a likely important factor in driving these aforementioned elevations in BMR. Increased thyroid activity may also play a part, especially in the longer-term elevation of BMR (see Chapter 16). Finally, it should be noted that any periods of intense activity are usually of short duration so that under normal conditions it is the BMR that is the largest contributor to TDEE. Given that much of acclimatization is a passive progress, and the obvious dangers of ascending too high too fast, the contribution of BMR to overall energy balance is particularly important. The BMR was found to be elevated in highland residents (Ladakhis and Sherpas) at high altitude compared with lowlanders (Gill and Pugh 1964; Nair et al. 1971), a result which persisted even when allowance was made for differences in body fat composition between the two groups. Picon-Reategui (1961) also reported elevated BMR in Andean miners at 4540 m. The mechanism for this difference in BMR between lowlanders and highlanders is uncertain but it would seem likely that, similar to lowlanders at high altitude, elevated sympathetic nervous and/or thyroid activity would likely play a key role for highlanders. The smallest component of TDEE in humans is food-induced thermogenesis, which is defined as the increase in metabolic rate observed for several hours following the ingestion of a meal (de Jonee and Bray 1997; Westerterp 2004). The thermic effect of food is believed to represent the energy cost of digestion, absorption, storage, and metabolic fate of dietary macronutrients (Westerterp 2004). While the precise mechanisms underlying the thermic effect of food are not fully understood, there is a clear macronutrient hierarchy, with protein causing a greater energy expenditure increment than carbohydrates, which is greater than that of fat (Westerterp 2004). For typical diet compositions, the thermic effect of food is approximately 10% of energy intake. Because energy and macronutrient intake are not particularly high at high altitude (see below), this expenditure likely accounts for less than 10% of the TDEE. Although maximum work rate is reduced following ascent (Chapter 18; Levett et al. 2011; Pugh 1964; West et al. 1983; Wolfel et al. 1991) and all activity is associated with increased breathlessness and perceived level of exertion compared to sea level, the daily energy expenditure is almost twice of that of normal daily expenditures at sea level (Figure 15.4) due to increased activity (e.g., multiple days trekking, etc.), increased work of breathing, and elevations in BMR. Even at altitudes of 2500–4500 m, it has been reported that the overall energy intake to maintain body weight increased from 13.22 MJ at sea level to 15.64 MJ a day at 4300 m due to the increase in BMR (Butterfield et al. 1992). Figure 15.4Daily energy expenditures (bars) and distances covered (circles) during a range of prolonged expeditions measured via doubly labeled water. Examples, from left to right, include climbing Mount Everest, unsupported crossing of the Antarctic, and elite endurance athletes racing or training. See references in text for further details. Before about 1990, it was impossible to measure energy expenditure over long periods, but a water labeling technique using both deuterium and 18O was developed that made this possible, though expensive. The deuterium is eliminated as water while the oxygen is eliminated as both water and carbon dioxide. Thus carbon dioxide production can be calculated from the different elimination rates (Coward 1991; Schoeller and van Santen 1982) and the daily energy expenditure can be estimated. Using this technique, Westerterp et al. (1992) found average daily energy expenditure in the Alps (2500–4800 m) and on Mount Everest (5300–8848 m) to be 15.7 MJ and 13.6 MJ, respectively. Very similar results were obtained in the 1992 British Winter Everest Expedition with values ranging from 11.7 to 15.4 MJ (Travis et al. 1993). Pulfrey and Jones (1996), using the same technique at altitudes of 5900–8046 m, found the very high mean values of 19.4 MJ day−1 and a negative energy balance of 5.1 MJ day−1. Reynolds et al. (1999) found the same high mean value of 20.6 MJ day−1 above base camp with a dietary intake of only 10.5 MJ, giving a deficit of 10 MJ day−1! Based on a chamber experiment simulating an ascent of Everest over 31 days (Operation Everest III), it was found that energy expenditure and BMR were more or less unaltered (Westerterp et al. 2000); therefore, it seems that the high altitude environment, that is to say, the combination of hypoxia, cold exposure, associated trekking, and food availability and palatability, rather than merely hypoxia per se, is necessary to induce the elevations in BMR and higher energy outputs found in field studies. Although the implications of a negative energy deficit are considered later in this chapter, the question arises: Are the energy expenditures while operating at very high altitude markedly higher than those seen with other extreme endurance activities? As illustrated in Figure 15.4, such energy expenditures, while almost double that of normal daily expenditures at sea level, are well below that of other pursuits such as the ski-crossing of the Antarctic (Halsey and Stroud 2012), ultra-endurance running (Eden and Abernethy 1994), cycling in the Tour de France (Westerterp et al. 1986), or cross-country ski training (Sjodin et al. 1994). Part of the difference may be attributable to the duration of time per 24 hours spent engaged in these activities. Time spent following safe ascent profiles and periods of acclimatization to attain moderate to high altitudes may be extended, but time spent at extreme altitudes is often short. Given the dangers of rapidly ascending to and climbing at extreme altitude, the distances covered are, by necessity, considerably less compared to these other activities at sea level. To this point in this chapter, we have established that body weight is reduced with prolonged exposure to high altitude, largely as a result of an accumulation of an energy deficit. A key question that remains is: Why does this deficit develop? Over the last 20 years or so, at least on commercial expeditions, food is generally in abundant supply. As a result, the well reported weight loss at altitude is most likely not due to the availability of food per se but rather due to other factors that cause decreased appetite and increased energy expenditures including neuroendocrine control of appetite, altitude illness, malabsorption, and/or impaired intestinal function. These factors are outlined next. Appetite is regulated, in part, by the neuroendocrine system (Murphy and Bloom 2006), and multiple hormones have been implicated as mediators of hunger and satiety in hypoxia (Bailey et al. 2000, 2004, 2015; Debevec et al. 2014, 2016; Matu et al. 2017; Shukla et al. 2005; Sierra-Johnson et al. 2008a; Tschöp et al. 1998), including glucagon-like peptide-1 (Bailey et al. 2004; Bailey et al. 2015), pancreatic polypeptide (Matu et al. 2017), peptide YY (Bailey et al. 2015; Wasse et al. 2012), neuropeptide Y (Vats et al. 2004), cholecystokinin (Aeberli et al. 2013; Riepl et al. 2012), adiponectin (Trayhurn, 2014), leptin (Tschöp et al. 1998), ghrelin (Benso et al. 2007; Mekjavic et al. 2016; Riedl et al. 2012; Riepl et al. 2012; Shukla et al. 2005), and insulin (Debevec et al. 2014; Matu et al. 2017; Mekjavic et al. 2016). The majority of research has focused on the latter three hormones and is discussed in more detail below. More detailed information can be found in several comprehensive reviews on this topic, including Debevec (2017) and Matu et al. (2018). The role of circulating leptin concentrations as a mediator of appetite and energy intake changes at altitude has been a topic of great interest and controversy. Leptin is an adipocytokine that has been proposed to express regulatory physiological effects on appetite and metabolism (Klok et al. 2007). It is well known that exposure to high altitude stimulates hypoxia-inducible factor 1 (HIF-1) and this can transactivate the human leptin gene promoter, potentially increasing circulating leptin concentrations (Grosfeld et al. 2002). On the contrary, altitude exposure is often associated with a significant loss of adiposity due to increased energy expenditure and/or decreased energy intake (see below), which would reduce leptin expression in adipose tissue. Consequently, the effects of high altitude exposure on leptin remain ambiguous (Sierra-Johnson et al. 2008b) with studies reporting increased (Tschöp et al. 1998), decreased (Bailey et al. 2004; Karl et al. 2018; Vats et al. 2004; Zaccaria et al. 2004), and unchanged (Smith et al. 2011) concentrations. It is now known that leptin release is influenced by several factors other than hypoxia (e.g., sleep, exercise, cold, adipose, and smoking status) and these may be confounding factors in some field studies. As highlighted in these studies and in a recent meta-analysis on the topic (Matu et al. 2018), it seems that leptin concentrations are not consistently affected by hypoxic exposure. As outlined in depth in Chapter 16, the effect of prolonged high altitude on plasma insulin concentration is variable, with studies finding reductions (Stock et al. 1978), no change (Bailey et al. 2004; Blume and Pace 1967; Debevec et al. 2014; Riedl et al. 2012), or elevations (Bosco et al. 2019; Siervo et al. 2014; Williams 1975; Young et al. 1989) in insulin concentrations. A recent meta-analysis (Matu et al. 2018) found a positive association between severity of hypoxic exposure and the magnitude of increases in insulin, and suggested that extreme altitude may account for the larger increases in insulin seen in some studies. To the extent that they do occur, increases in insulin concentration may contribute to the observed reductions in hunger during hypoxic exposure but could also represent a reduction in insulin sensitivity (Karl et al. 2018; Woolcott et al. 2015). An increase in fasting, but not postprandial insulin concentrations, suggests that hepatic insulin sensitivity is more heavily influenced by hypoxia than peripheral insulin sensitivity (Radziuk 2014). The use of a hyperinsulinemic, euglycemic clamp in humans has demonstrated that an acute 30-minute hypoxic exposure (resulting in a blood oxygen saturation of ∼75%) rapidly reduces whole-body insulin sensitivity by ∼15% (Oltmanns et al. 2004). The reduction in insulin sensitivity could be due to catecholamine responses, adipose tissue inflammation, and/or HIF signaling (Murphy et al. 2017; Oltmanns et al. 2004). However, further work is required to establish whether chronic, sustained hypoxia reduces tissue insulin sensitivity and has any neuroendocrine role in appetite control. Acylated ghrelin has been hypothesized to act physiologically to signal hunger and initiate eating and has received growing attention in hypoxic research during recent years (Bailey et al. 2015; Goto and Morishima 2016; Karl et al. 2018; Matu et al. 2017; Wasse et al. 2012). Current evidence suggests that appetite and acylated ghrelin are concomitantly suppressed during exposure to high (>3500 m), but not moderate, simulated altitude. Based on these data and those outlined below, a potential mediating role of acylated ghrelin in high altitude-induced anorexia has been proposed (Karl et al. 2018; Matu et al. 2017). However, due to the complex chemical preparation required for accurate acylated ghrelin measurements, total ghrelin concentrations have been more commonly measured in response to hypoxic exposure. A recent meta-analysis on the topic found a positive association between hypoxic severity and the decrease in acylated ghrelin concentrations. Specifically, it was demonstrated that acylated ghrelin is suppressed in hypoxia compared with normoxia but that total ghrelin concentrations remain unchanged. Such findings are consistent with those of Matu et al. (2017), who found that acylated ghrelin was suppressed with high but not moderate simulated altitude exposure. Total ghrelin consists of the combined levels of des-acyl ghrelin and acylated ghrelin, and recent research has found that des-acyl ghrelin can inhibit the orexigenic effects of acylated ghrelin by targeting the arcuate nucleus, independently of the growth hormone secretagogue receptors (Fernandez et al. 2016). The opposing effects of these hormones suggest that physiologically relevant changes in ghrelin constituents may be masked by the measurement of total ghrelin. It would therefore be beneficial for further research in this area to differentiate between the ghrelin constituents. Overall, it seems that postprandial acylated ghrelin concentrations are generally decreased during hypoxic exposure compared with normoxia. The observed reductions in hunger and energy intake are in accord with the hypothesized orexigenic effects of acylated ghrelin (Monteiro and Batterham 2017). In part due to a lack of research, the influence of high altitude on other hormones related to appetite suppression is less clear. On first arrival at high altitude, acute mountain sickness (AMS) may cause anorexia and vomiting with resultant weight loss. However, fluid can often be retained, causing those suffering AMS to gain weight (Hackett et al. 1982). Consolazio et al. (1972) found a small gain in weight on the first day at high altitude in those with mild AMS followed by a loss of weight of about 1 kg over the next five days at 4300 m. The mechanism(s) of the anorexia as a symptom of AMS is not clear (Debevec 2017). Loss of appetite due to AMS is unlikely to be the sole cause of weight loss, as symptoms of anorexia persist even after AMS has subsided (Tschöp and Morrison 2001) and others have found appetite suppression in individuals without symptoms of AMS (Matu et al. 2017). In addition to AMS, other forms of illness seen among high altitude travelers, including gastrointestinal and upper respiratory tract infection, are important factors that can directly and indirectly lead to weight loss while at high altitude. Anorexia might occur if exposure to hypoxia at high altitude blunts the normal increase in gastrointestinal blood flow. This possibility was tested in a study by Kalson et al. (2010) who used ultrasound to measure velocity and vessel diameter of the hepatic portal vein (as an index of flow from the gut to the liver) in 12 subjects before and after a meal at sea level and various altitudes up to 3767 m. They found increased flow at altitude but the increase in postprandial blood flow was still maintained. Although this finding suggests that inadequate increase in blood flow from the gut is not a cause of altitude anorexia, other indirect evidence suggests that gut mucosal blood flow may be altered in hypobaric hypoxia. For example, a study of Qinghai railroad construction workers showed a 0.5% incidence of life-threatening gastrointestinal bleeding due to ulcer formation (Wu et al. 2007). Broadly consistent with this observation, in 14 of 23 (61%) healthy mountaineers staying their fourth night at 4559 m, the presence of peptic mucosal lesions such as erosions, ulcers, and hemorrhagic gastritis/duodenitis was observed (Fruehauf et al. 2019). Such lesions may be the result of alterations in gut mucosal blood flow, as can be seen in patients with critical illness and impaired tissue oxygen delivery. Given that weight loss occurs at altitudes above 5000 m with, in some cases, adequate intake and reduced energy output, the possibility of malabsorption of food must also be considered. In this context, malabsorption is defined as a situation in which the small intestine is unable to absorb appropriate amounts of delivered nutrients and/or fluids. Pugh (1962) reported that members of the Silver Hut expedition reported greasy and bulky stools, suggesting possible steatorrhea due to malabsorption of fat. There seems to be little work carried out on this topic, perhaps because the methods involved are either too sophisticated for easy use in the field (e.g., absorption of radioactive materials) or are unattractive to investigators (e.g., fecal collection, liquidization and aliquot sampling, etc.) or because few altitude physiologists have a background or training in gastroenterology. The following sections review the limited number of studies that have assessed carbohydrate, fat, and protein absorption at high altitude. In a field study, Chesner et al. (1987) found no malabsorption of xylose in 11 subjects up to 4846 m. However, 60-minute plasma xylose (5-carbon monosaccharide) concentrations were reduced in subjects who ascended to 5600 m, suggesting that absorption is not affected until hypoxia is severe. Boyer and Blume (1984), who studied subjects at 6300 m, found xylose absorption decreased by 24% in six out of seven subjects, compared with sea-level controls. However, absorption measured by xylose has the drawback that the result is influenced by factors such as gastric emptying time, absorption area, intestinal transit, and renal function. Dinmore et al. (1994) used a double carbohydrate test; the two nonmetabolized carbohydrates used undergo different forms of mediated absorption but are otherwise subject to the same external influences, which cancel out when results are expressed as a ratio (Menzies 1984). D-xylose is absorbed by passive mediated transport, whereas 3-O-methyl-d-glucose is absorbed by active mediated, sodium-dependent transport. Dinmore et al. (1994) found that at 6300 m there was a 34% decrease in d-xylose (Figure 15.5) and a 15% decrease in 3-O-methyl-d-glucose absorption. The ratio was consistently decreased at altitude, and in a subsequent study, the 60-minute serum xylose/3-O-methyl-d-glucose ratio was 17% lower at 5400 m than at sea level (Travis et al. 1993). These more sophisticated studies therefore support the hypothesis that at these high altitudes carbohydrate absorption is impaired (reviewed in: Hamad and Travis 2006). Figure 15.5d-xylose absorption tested in a group of climbers at sea level (UK), at altitudes indicated in Nepal, and after return to the UK (with mean and SD values at each location). (Data from Dinmore et al. 1994.) The majority of studies have not found evidence of fat malabsorption at high altitude. For example, Rai et al. (1975) found no evidence of fat malabsorption at 4700 m, as did Chesner et al. (1987) at 3100 m and 4800 m. Imray et al. (1992) used the 14C-triolein breath test and found no malabsorption of fat at 5500 m on Aconcagua, while Butterfield et al. (1992) found no increase in fecal excretion of volatile fatty acids at 4300 m. In contrast to these studies, Boyer and Blume (1984) found that fat absorption decreased by 49% at 6300 m compared with sea level in three acclimatized subjects. Differences in altitudes and the techniques to measure fat absorption likely explain these contradictory findings. Kayser et al. (1992) measured protein absorption using urinary and fecal 15N excretion after ingestion of 15N-labeled soya protein and found no reduction in absorption in subjects after three weeks at 5000 m. The observation is consistent with a more recent report showing that a high protein diet did not influence weight loss over 22 days at high altitude when compared with an isoenergetic standard diet (Berryman et al. 2017; see Figure 15.6). Figure 15.6Mean change in body mass with consumption of an isoenergetic standard protein (SP: N = 8) vs. a high protein (HP: N = 9) diet during energy deficit at 4300m. *P <0.05 vs. SL (sea level). There were no differences between diets. (From Berryman et al. 2017.) In summary, there is little evidence of malabsorption up to an altitude of about 5000 m. This has been confirmed by measurements of fecal energy excretion, which have shown that 96% of energy intake is assimilated (Kayser et al. 1992), a normal sea-level value for subjects on a Western low residue diet. There is always the possibility that intestinal infections, at the time of the study or in the previous few days or weeks, may have caused some malabsorption since many of these field studies were undertaken in countries where such infections are all too common. The obvious muscle wasting seen especially in climbers returning from extreme altitude prompts the question of whether hypoxia affects protein turnover directly. There are very few data on this topic in humans. Consolazio et al. (1968) first studied protein balance at high altitude and found no difference between subjects there and at sea level, but the altitude station was Pikes Peak (4300 m), below the crucial height at which continued weight loss is observed. Rennie et al. (1983) studied the effect of acute hypoxia in a chamber (equivalent altitude 4550 m) on leucine metabolism in forearm muscles and found that acute hypoxia resulted in a net loss of amino acids from the muscles, probably due to a fall in muscle protein synthesis. If this finding can be extrapolated to the situation of chronic hypoxia at altitudes of above 5000–6000 m, then it provides a further contributing factor to the loss of muscle mass described above. As such, at least at sea level, higher protein (>1.2 g of protein per kg of body weight per day) diets preserve fat-free mass during weight loss (Leidy et al. 2015; Pasiakos et al. 2014; Wycherley et al. 2012), which may be beneficial for maintaining physical function and health. These benefits underpin recommendations supporting higher protein diets for athletes (Thomas et al. 2016) and military personnel (Pasiakos et al. 2017, 2013, 2014, 2015) and have stimulated recent interest in studying the effectiveness of higher protein diets for mitigating fat-free mass loss during high altitude sojourn (Pasiakos et al. 2017). However, protein increases fullness (Dhillon et al. 2016) and is more satiating than carbohydrate or fat (Leidy et al. 2015). These effects may be potentiated at high altitude due to the higher thermogenic effect of protein relative to fat (Veldhorst et al. 2008), which could worsen hypoxia by increasing postprandial oxygen consumption (Westerterp and Kayser 2006). These observations are consistent with a recent report that consuming a controlled higher protein hypocaloric diet relative to a controlled standard-protein hypocaloric diet did not protect fat-free mass during weight loss over 22 days at 4300 m (Berryman et al. 2017). The likely mechanism to explain the lack of influence of the high protein diet in preventing weight loss was due to an enhanced protein oxidation. Appetite-mediating hormones (e.g., insulin, leptin, and cholecystokinin and acylated ghrelin) as well as subjective reports of appetite and satiety were also not different between the high and low protein diets (Karl et al. 2018). Given the prevalence of weight loss among high altitude travelers, particularly at the extremes of elevation, a common consideration is whether anything can be done to combat these changes. An obvious area of intervention would be to manipulate dietary intake. Views on diet are strongly held, but often the strength of opinion is disconnected from the strength of scientific evidence. Fundamentally, however, since marked negative energy balance can lead to accentuated catabolism (D’Hulst and Deldicque 2017), immune suppression (Hartmann et al. 2000), and oxidative stress (Lewis et al. 2014), the dietary goal of maintaining energy balance (via energy intake) should be prioritized. Despite several studies that have explored the merit of manipulation of carbohydrate or protein intake at high altitude, no approach has yet to be effective in attenuating weight loss or improving physical performance. The evidence for each of the main approaches is outlined below. For optimizing performance at sea level during moderate to high-intensity exercise, the evidence overwhelmingly supports the inclusion of a moderate-to-high carbohydrate diet (i.e., ∼60% of energy intake, 5–8 g of carbohydrate per kg of body weight per day) to mitigate the negative effects of chronic, training-induced glycogen depletion (Burke and Hawley 2018; Hargreaves and Spriet 2018; Hearris et al. 2018). Likely because of this approach at sea level, there is sometimes a preference among climbers for a high carbohydrate, low fat diet at altitude. Although there is little evidence of glycogen depletion at high altitude, Figure 15.7 shows some of the theoretical basis for advising a high carbohydrate diet, which moves the respiratory quotient (RQ) from 0.7, if one uses fat exclusively for energy, to 1.0 when carbohydrate is used. The result of such a change of RQ is that for any given PACO2 the PAO2 is increased. In the case illustrated in Figure 15.7, the subject is considered to be at 5800 m when the barometric pressure is half that at sea level and the PI
Introduction
Magnitude of Weight Loss at High Altitude
Moderate altitude (1500–3500 m)
High altitude (3500–5300 m)
Extreme altitude (>5300 m)
Sex differences in weight loss at high altitude
Weight loss in chamber experiments
Body Composition During Weight Loss at High Altitude
Changes in body composition during chamber studies
Energy Deficit at High Altitude
Determinants of energy balance
Total daily energy expenditure
Basal Metabolic Rate in Lowlanders Ascending to High Altitude
Basal Metabolic Rate in Long-Term High Altitude Residents
Diet-induced energy expenditure
Activity-induced energy expenditure
Mechanisms for the Energy Deficit and Weight Loss
Neuroendocrine control of appetite at high altitude
Leptin
Insulin
Ghrelin
Other factors affecting weight loss at high altitude
Altitude Illness
Gastrointestinal Blood Flow
Gastrointestinal Absorption
Carbohydrate absorption
Fat absorption
Protein absorption
Changes in Protein Metabolism
Diet for High Altitude
High carbohydrate diet
Stay updated, free articles. Join our Telegram channel
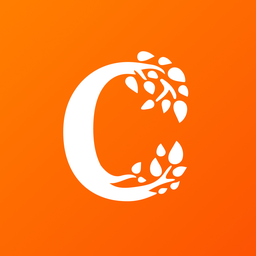
Full access? Get Clinical Tree
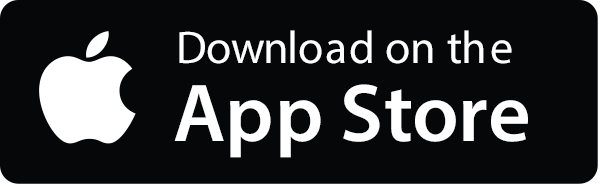
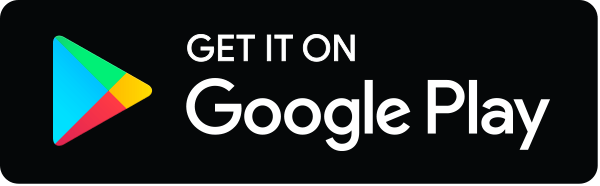