Hormones involved in salt and water regulation While the topics of endocrinology and metabolism involve many systems controlling a great variety of bodily functions, the effect of altitude has been studied on only a fraction of these. The areas studied reflect the interests of scientists in understanding the endocrinologic and metabolic challenges to high altitude, because these issues not only affect well-being at high altitude but also have important implications for those traveling to altitude with various endocrine and metabolic disorders. Among those most studied are hormones that affect the salt and water balance of the body and regulate cardiovascular function. Moreover, hormones affecting fluid and electrolyte balance have been widely studied because of their possible relevance to acute altitude illness, while thyroid hormones have been studied because of their effects on metabolic rate. Extended residency at high altitude can lead to long-term hematologic and other disorders, such as excessive erythrocytosis (EE) and pulmonary hypertension, which partly result from hormonal maladaptation to altitude. Because exercise affects many hormonal systems and is an essential activity at altitude, it must also be considered as part of a comprehensive examination of endocrine and metabolic responses. This chapter surveys the principal changes in hormonal balance and metabolism upon initial arrival and with acclimatization to high altitude. To provide a framework for the concepts covered in this chapter, Table 16.1 summarizes the main hormones that are considered below, including their responses and function, and the influence of high altitude or exercise. Table 16.1 Summary of the main hormones outlined in this chapter, including their responses and function, and the influence of high altitude or exercise As discussed further in Chapter 20, there has been evidence that ascent to altitude is associated with changes in body fluid compartments and that interindividual variability in such responses may affect susceptibility to acute mountain sickness (AMS). For this reason, a considerable number of investigations have examined various hormones involved in salt and water regulation and how production and activity of these hormones change with exposure to hypoxia. Each of these major hormones is considered further below. Antidiuretic hormone (ADH), also known as arginine vasopressin (AVP), is secreted by the posterior pituitary in response to rises in plasma osmolality and has the effect of increasing water reabsorption in the renal collecting ducts, thus reducing urine volume. In some animals, it also has an effect on arterioles and contributes to blood pressure regulation, hence its alternative name, but this effect is minimal in humans. Forsling and Milledge (1977) found that breathing 10–10.5% oxygen for four hours had no effect on ADH concentrations, while in a subsequent chamber experiment, where subjects were exposed to an inspired oxygen fraction equivalent to 4000 m for 14 hours, there was no significant change in the plasma ADH concentration until subjects began to feel nauseated, after which time the concentration rose markedly (Forsling and Milledge 1980). Claybaugh et al. (1982) took subjects to various equivalent altitudes in a hypoxia chamber and found an initial increase of urinary ADH over eight to 12 hours of hypoxia with subsequent return to sea-level values within a day. In two subjects with AMS, there was a rise in urinary excretion of ADH at two to four hours of hypoxia. De Angelis et al. (1996) studied 26 young pilots in a chamber at an equivalent altitude of 5000 m for three hours and found a significant increase in ADH as a result of this quite severe hypoxic stress. The conclusion from a number of early studies that collected blood samples at various altitudes (4300–5400 m) was that there is no significant effect on ADH concentration (Cosby et al. 1988; Hackett et al. 1978; Harber et al. 1981; Ramirez et al. 1992; Singh et al. 1974). There was some evidence from these studies that ADH may be higher as a consequence (rather than cause) of AMS or high altitude pulmonary edema (HAPE); however, this is not a universal finding. Consistent with the observations, Loeppky et al. (2005) studied 51 men in a chamber at 4880 m equivalent altitude over eight to 12 hours and measured fluid balance, various hormones, and AMS scores. They found that those with the most severe AMS had more fluid retention and lower urine output compared to those without AMS. Specifically, the AMS group had a positive balance of 1197 mL while the non-AMS had a net fluid balance of –724 mL, a difference of almost 2 L. ADH rose in those with AMS and fell in those with little or no AMS. This result suggests that the ADH responses may affect susceptibility to AMS. Those who remain well experience water diuresis due to a fall in ADH level while those individuals in whom ADH fails to decrease are more likely to get AMS. It must be recognized, however, that rather than being part of a causal pathway, the observed changes in ADH may be an epiphenomenon of AMS (see Chapter 20 for further details). In the context of longer sojourns at altitude, a recent study reported significantly decreased ADH at 3440 m and 5050 m, both on arrival and after 14 days, in 28 volunteers (Haditsch et al. 2015). Interestingly, there also seemed to be a sex difference in some of these hormonal responses (Haditsch et al. 2015). A significant decrease in ADH was found in males at both altitudes but only at the higher elevation in females. At least in this study, the reduction in ADH during ascent and over time at altitude was accompanied by diuresis and a decrease in urinary osmolality. Studies on the effects of chronic hypoxia on ADH are equivocal. When HAPE and AMS do not develop, ADH also does not seem to rise. This would seem to imply that ADH and plasma osmolality, which plays a role in high altitude edema, are linked. The relationship between plasma osmolality and ADH concentration is discussed below. Blume et al. (1984) presented evidence of inappropriately low secretion of ADH at altitude. They studied 13 subjects after some weeks at 5400 m and 6300 m on Everest during the American Medical Research Expedition to Everest (AMREE) in 1981 and found ADH concentration unchanged from sea level despite a significant increase in plasma osmolality with increasing altitude. At 6300 m, the serum osmolality was 302 mOsm·kg−1 compared with 290 mOsm·kg−1 at sea level (normal value 280–295 mOsm·kg−1). An overnight dehydration test at sea level that might produce this degree of hyperosmolality would result in ADH concentrations of about 7 µU·mL−1, whereas subjects on Everest had a mean value of only 0.9 µUmL−1; 12-hour urinary ADH showed the same lack of response. Sodium, potassium, calcium, and phosphate concentrations were all modestly increased compared with sea-level values. A study by Ramirez et al. (1992) confirmed these observations. Here, osmolality was increased by infusion of intravenous sodium chloride (0.9%), both at sea level and at altitude (3000 m). At sea level there was the expected rise in ADH but at altitude there was no significant rise. Thus, at altitude, there seems to be a failure of the osmoregulatory mechanism(s). A later study (Ramirez et al. 1998) found evidence of reduced sensitivity of the kidney to ADH in acclimatized individuals and that infusion of exogenous ADH caused an increase of urinary ADH-sensitive water channel (aquaporin-2). Maresh et al. (2004) explored the response in ADH secretion to a water deprivation test at sea level, on acute altitude exposure (two days) and more chronic exposure (20 days) on Pikes Peak (4300 m). Similar to other studies, they found higher plasma osmolality at altitude but no significant change in baseline levels of ADH. With water deprivation, the rise in ADH was greater at altitude, and more so on day 20 than at day 2 of their altitude stay, and the osmolality threshold which stimulated ADH release appeared to rise (Figure 16.1). An eight-day study by Bestle et al. (2002) at the Capanna Margherita (4559 m) using a hypertonic saline (5% hypertonic saline; 3.6 mL/kg over one hour) loading test found the ADH response unchanged from sea level. Both studies found the function curve of osmolality and ADH shifted to the right with ascent; that is, the set point of plasma osmolality to ADH level is elevated at altitude and continues to increase with time at altitude. Since Maresh et al. (2004) found higher baseline levels of ADH, they also showed higher osmolality at two days and more so after 20 days spent at 4300 m. Figure 16.1Regression analysis describing plasma osmolality (Posm) versus plasma antidiuretic hormone (ADH) forced into a linear model. Solid regression line, SL, dotted line, two days at altitude, dashed line, 20 days at altitude. (Source: Maresh et al. 2004 with permission.) Note how a given ADH concentration requires a higher osmolality with increased altitude. These results suggest that elevations in resting osmolality occur as the result of altitude-induced reductions in plasma volume (Maresh et al. 2004). Alternatively, blood pressure or plasma volume increases may also cause changes in the ADH-osmolality relationship via baroreceptor stimulation in the circulatory system. The fact that ADH concentration is reduced despite increases in osmolality (Bestle et al. 2002) could be a consequence of baroreceptor mediated changes in the relationship. Figure 16.2 illustrates the changed set point of the ADH response to changes in osmolality following ascent, and how higher plasma osmolality elicits higher ADH concentration after acclimatization. Figure 16.2Effects of high altitude on plasma osmolality (⦁, ▴) and plasma ADH concentration (⚬, △) over time. ▴ and △ from Maresh et al. (2004) baseline prior to water deprivation tests at sea level (SL) and after two and 20 days at 4300 m (N = 7). ⦁ and ⚬ from Bestle et al. (2002) baseline prior to hypertonic load at sea level and one, two, three, six, seven, and eight days at 4559 m (N = 8). Note Maresh et al. found higher osmolality and ADH, demonstrating the shift in the response point of ADH to higher osmolality. The renin-angiotensin-aldosterone (RAA) system is a complicated system of reactive and compensatory mechanisms involving by renin, angiotensinogen, angiotensin I and II, angiotensin-converting enzyme, and aldosterone that regulates fluid and sodium balance and blood pressure in both health and disease (Figure 16.3). Renin is released in response to a number of stimuli, including posture, exercise, and, possibly, hypoxia. Because sympathetic activation is a common response across all of these stimuli and acute hypoxia increases both circulating catecholamines and direct sympathetic nervous activity (Hansen and Sander 2003; Simpson et al. 2019), high altitude exposure leads to release of renin from the juxtaglomerular apparatus of the kidney. Figure 16.3Renin-angiotensin-aldosterone system. Renin and angiotensin-converting enzyme (ACE) hydrolyze angiotensinogen and angiotensin I to angiotensin II. The latter stimulates release of aldosterone from adrenocortical cells by type 1 and type 2 angiotensin II receptor mechanisms, and via adrenocorticotropic hormone (ACTH) secretion. High altitude, the hypoxic environment in particular, downregulates both renin and aldosterone activity. This contributes to high altitude diuresis and natriuresis by reducing aldosterone-mediated H2O and Na+ reabsorption. Furthermore, hypoxia dissociates renin from angiotensin II via downregulated ACE activity (Vonmoos et al. 1990). In cases where this does not occur, overactive angiotensin II mediated vasoconstriction may contribute to heightened pulmonary hypertension and HAPE. Renin has little biological activity but act on its circulating substrate, angiotensinogen, cleaving it to produce the octapeptide angiotensin I, which is also devoid of substantial activity. Angiotensin-converting enzyme (ACE), found on the luminal surface of endothelial cells, converts angiotensin I to angiotensin II by cleaving the final two amino acids with the primary site of such conversion being the rich capillary network of the lung, where nearly 90% of angiotensin I is converted to angiotensin II in a single passage. Angiotensin II is a powerful vasopressor and acts on the glomerulosa cells of the adrenal cortex via AT1 and AT2 receptors to release aldosterone, which subsequently acts on the renal tubules to cause sodium reabsorption. Because the system is heavily involved in salt and water regulation, it has also been the subject of investigation at high altitude. Most studies have demonstrated a reduction in plasma renin activity (PRA) at rest with high altitude exposure (Bärtsch et al. 1988; Bärtsch et al. 1991b; Bestle et al. 2002; Haditsch et al. 2015; Keynes et al. 1982; Olsen et al. 1992; Zaccaria et al. 1998), as well as reductions in resting aldosterone concentrations (Bärtsch et al. 1988; Bärtsch et al. 1991b; Keynes et al. 1982; Loeppky et al. 2005; Milledge et al. 1983b; Zaccaria et al. 1998) (Figure 16.4). A relatively greater drop in plasma aldosterone concentration to plasma renin activity increases the renin:aldosterone ratio, indicative of a reduced aldosterone response to renin. This may be partly due to direct inhibition of aldosterone synthesis in the adrenal cortex by hypoxia (Raff et al. 1996). Figure 16.4Relative changes in basal plasma renin activity (PRA) compared with relative changes in plasma aldosterone concentration (PAC) at altitude. Note: most AMS values are above non-AMS. Specific studies are noted on the graph. General relationship of PAC and PRA depicted (R2 = 0.3). (Source: Bärtsch et al. 1988; Bärtsch et al. 1991a; Bestle et al. 2002; Haditsch et al. 2015; Loeppky et al. 2005; Milledge et al. 1983b; Olsen et al. 1992; Zaccaria et al. 1998.) As aldosterone has a primary role in water and sodium reabsorption, this reduction in plasma aldosterone concentration is a beneficial adaptation that facilitates the natriuresis and diuresis required for the initial hemoconcentration upon exposure to high altitude. Indeed, it is a relatively consistent finding that, in the absence of such a response, plasma aldosterone is elevated and potentially contributes to increased fluid retention and AMS (Bärtsch et al. 1991b; Bärtsch et al. 1991a) (Figure 16.4). In addition to regulation by renin/angiotensin II, aldosterone concentration is also controlled by adrenocorticotropic hormone (ACTH) and the sodium/osmolality status of the subject. For example, Ramirez et al. (1988) found significant inhibition of aldosterone secretion in hypoxia in both lowlanders at sea level and highlanders at altitude, including a blunted aldosterone response to exogenous ACTH, possibly due to increased atrial natriuretic peptide (ANP; see ANP section below), whereas ACTH-induced secretion of cortisol was unaffected (see: Corticosteroids at altitude below). Since exercise is commonly performed as part of ascent to high altitude, the effect of exercise must be considered in relation to the effect of altitude on the RAA system (Table 16.1). Exercise stimulates renin release via activation of the sympathoadrenal system, with the rise in PRA being proportional to the intensity of the work, both at sea level and at altitude (Maher et al. 1975a). After intense short-term exercise at sea level (3 × 300 m sprints in 10 minutes), PRA, angiotensin II, and aldosterone concentration are significantly elevated at 30 minutes by 108% (27–230%), 830% (400–1970%), and 1600% (160–3920%), respectively (means and ranges). This effect is present up to six hours later (Kosunen and Pakarinen 1976) and can be attenuated by administration of beta-blockers (Bonelli et al. 1977; Bouissou et al. 1989). In a between-groups chamber study, aldosterone levels were raised in both normoxia and acute normobaric hypoxia (FIO2 0.11) immediately after 90 minutes of graded submaximal treadmill exercise. Of note, aldosterone concentrations were significantly greater in the normoxic control group than the hypoxia group both immediately following exercise and after 60 minutes of rest (Cooke et al. 2018). No differences were found in angiotensin-converting enzyme, aldosterone synthase, potassium, or serum osmolality, indicating that the blunting of aldosterone secretion in hypoxia is not a consequence of reductions in angiotensin- or potassium-mediated mechanisms. Mountaineering involves day-long exercise rather than acute bouts, often continuing for a number of days. Williams et al. (1979) showed that this form of exercise resulted in marked sodium retention after 7 days and suggested that this was due to activation of the RAA system. There was a mean cumulative retention of 358 mmol of sodium with a modest retention of 650 mL of water. Since plasma sodium concentration did not change significantly, it was argued that the extracellular space must have been expanded by 2.68 L (of which 0.68 L was in the plasma volume), mainly at the expense of the intracellular volume (see Figure 13.2). This increase in extracellular fluid is the probable cause of the dependent peripheral edema frequently found after exercise of this sort. In a study of the effects of five consecutive days trekking at low altitude (<1500 m), Milledge and Catley (1982) also found that the sodium retention was associated with activation of the RAA system, and PRA and aldosterone were elevated at the conclusion of each day’s exercise and returned to baseline levels two days after cessation of exercise. The effect of exercise and altitude was studied by repeating the same protocol, but on the first exercise day, subjects climbed to 3100 m and stayed there for five days, exercising for eight hours each day. The results were very similar to sea-level results in terms of changes in fluid and sodium balance and hematocrit. Renin and aldosterone also increased, but the aldosterone response to the increase in renin was blunted (Milledge et al. 1983c). Milledge and Catley (1982) showed that if after one hour of exercise the fraction of inspired oxygen was reduced, renin activity increased while aldosterone concentration decreased, indicating that the aldosterone response to renin was blunted. The same phenomenon is seen with states of chronic exercise such as trekking or climbing at altitude, as demonstrated in Figure 16.5, which shows data from studies performed at sea level, 3100 m, and 6300 m. This blunting of the aldosterone response to renin in acute hypoxia has been confirmed in several other studies. Shigeoka et al. (1985) found the response completely abolished at a FIO2 of 0.17, while Lawrence et al. (1990) found decreased aldosterone with unchanged PRA at a FIO2 of 0.12 and De Angelis et al. (1996) demonstrated increased PRA and decreased aldosterone at a simulated altitude of 5000 m in a hypobaric chamber. Additionally, Li et al. (2016) studied 138 men following rapid ascent to 3450 m and found the aldosterone response to renin was attenuated by administration of budesonide, more so than with dexamethasone or placebo. Based on this result, the authors hypothesized that the budesonide-induced dissociation of aldosterone concentration from PRA could explain the observation in some studies that budesonide was effective at preventing AMS (Chen et al. 2015; Zheng et al. 2014). Other studies (Berger et al. 2018; Lipman et al. 2018) have not shown any effect of budesonide on AMS prevention, however, and, as a result, the significance of the observed effect of budesonide on the aldosterone response to renin remains unclear. Figure 16.5Plasma aldosterone concentration (PAC) response to plasma renin activity (PRA) from a high altitude study (6300 m) (individual data before and after exercise) and from two separate studies (sea level and 3100 m) (mean data from five and six subjects). (Source: Milledge et al. 1983a.) Aside from its effects on sodium and water balance, the RAA system also has effects on systemic blood pressure, at least at sea level. However, while suppression of angiotensin II and angiotensin-converting enzyme has been shown to be a significant contributor to overall suppression of the RAA system with acute exposure to high altitude, pharmacological inhibition of the RAA system with angiotensin receptor antagonists is not effective at reducing hypertension at very high altitudes (Parati et al. 2014; Revera et al. 2017). Atrial natriuretic peptide (ANP) is secreted by the atria of the heart in response to stretching rather than simply changes in pressure (Au et al. 1990). Among its actions, ANP has the effect of increasing renal sodium excretion and, therefore, promoting natriuresis and diuresis (Morice et al. 1988). When plasma volume rises, the increase in atrial pressure causes atrial stretch and secretion of ANP, which subsequently leads to diuresis and a decrease in intravascular volume and pressure. This system is further considered in relation to the regulation of plasma volume in Chapter 13. Atrial natriuretic peptide may also have vasodilatory properties and, in particular, blunt the effect of hypoxia on pulmonary arterioles. For example, dose-dependent pulmonary vasodilation has been demonstrated in an isolated rat lung (Stewart et al. 1991) and in pigs (Adnot et al. 1988), while ANP knock-out mice have greater remodeling of the pulmonary arteries in chronic hypoxia when compared to wild-type controls (Chen et al. 2006). Similarly, Liu et al. (1989) infused ANP (20 mg min−1 for 10 minutes) into four patients with HAPE and demonstrated a reduction in pulmonary artery pressure for one hour after the infusion. A variety of studies in animals and humans have examined the effect of hypoxia on plasma ANP concentrations at rest and with exercise. Ten minutes of severe hypoxia on isolated rat and rabbit heart with constant flow perfusion caused a four-fold increase in ANP released (Baertschi et al. 1986). The same group found increases in ANP concentration in whole animals exposed to hypoxia during anesthesia (Baertschi et al. 1988). There was great variability in the observed responses, which correlated with the baseline central venous pressure, but not with any other measured variables. Figure 16.6 summarizes the observed changes in ANP concentration in animal studies in hypoxia. Figure 16.6Comparative percent changes of atrial natriuretic peptide (ANP) from animal-based hypoxia studies. Baertschi et al. (1986): isolated rat hearts (left) and isolated rabbit hearts (middle), 10 mins of PO2 21 ± 4 mmHg, post ANP at eight minutes post hypoxia. Baertschi et al. (1988): rabbits, 10 minutes of PO2 22-44 mmHg, post ANP at 8 minutes post hypoxia. Collectively, these data indicate a marked rise in plasma ANP during reduced PO2 and a compensation following reoxygenation in animal models. In humans, Kawashima et al. (1989) studied the effect of 10 minutes of hypoxia at two levels, an FIO2 of 0.15 and 0.10. Exposure to the former produced no change in ANP concentration while exposure to the latter increased ANP levels by 15% and also increased pulmonary artery pressure. Vonmoos et al. (1990) found that 60 minutes of breathing a hypoxic gas mixture (FIO2 0.12) produced a small but significant elevation of ANP. Lawrence et al. (1990) found that the same hypoxic stimulus produced a 50% increase in ANP concentrations in subjects on a low salt diet and whose endogenous cortisol was suppressed with dexamethasone. Conversely, Ramirez et al. (1992) did not find a significant rise in ANP concentration with either acute (60 minutes) or chronic exposure to 3000 m, although the ANP response to a sodium load was greater at altitude. Antezana et al. (1995) found a reduction in ANP concentration in lowlanders at 3600 m compared with sea-level values and also reported lower concentrations in highlanders when compared to the lowlanders at high altitude. Figure 16.7 demonstrates the observed changes in ANP concentration in humans exposed to hypoxia. Figure 16.7Comparative percent changes of atrial natriuretic peptide (ANP) from human studies in hypoxia. Ramirez et al. (1988): two hours FIO2 0.13, administered with dexamethasone and incrementally dosed with ACTH throughout hypoxia, post measure = end of hypoxia. Kawashima et al. (1989): 10 minutes FIO2 0.10 (BA, brachial artery; PA, pulmonary artery), only pulmonary ANP change was significant, no post measures. Lawrence et al. (1990): 60 minutes FIO2 0.12, subjects on low salt diet and dexamethasone. Vonmoos et al. (1990): 60 minutes FIO2 0.12, peak ANP at 45 minutes significantly different to control ANP, 15 minutes after metoclopramide administration, post ANP at 45 minutes posthypoxia. Collectively, these data indicate a rise in plasma ANP in humans during hypoxia similar to those in previous animal models (Figure 16.6), as well as increased ANP responsiveness to hypoxia with dexamethasone. The discrepancies in results between these studies may be explained by differences in study protocols and, in particular, the extent of hypoxia used. For example, Kawashima et al. (1989), Vonmoos et al. (1990), and Lawrence et al. (1990) found ANP increased using FIO2 <0.12, while Ramirez et al. (1992) and Antezana et al. (1995) studied hypoxia at <3600 m (FIO2 ∼ 0.13) and showed no change or a slight decrease in ANP concentration. This suggests that the severity of hypoxia affects the extent of hemodilution and the subsequent effects on atrial-stretch and ANP release. This notion is further supported by evidence that hypoxemia itself is a direct stimulus to ANP secretion even in the absence of atrial stretch due to hypoxia-induced hemodilution. For example, in isolated mouse atrial myocytes, Chen et al. (1997) showed that severe hypoxemia (cell media 1% O2, PO2 20–30 mmHg) is a strong stimulus for ANP gene expression that peaks after five days. It is well established that ANP increases with exercise in athletic, control, and pathophysiological humans (Barletta et al. 1998; Nielsen et al. 2001; Steele et al. 1997; Wisén et al. 2011), and it has been shown that ANP release is influenced by exercise intensity; higher intensities cause increased atrial stretch (Barletta et al. 1998) (Figure 16.8). Somers et al. (1986) found that a progressive exercise test to maximum exercise resulted in an almost four-fold increase in plasma ANP with a decline to baseline after one hour at rest. Similar results have been found for short-term exercise by Schmidt et al. (1990) and by Lawrence and Shenker (1991). Trekking at low elevations (<1500 m) for five days also resulted in elevated ANP concentrations to about twice baseline values (Milledge et al. 1991). It is interesting to note that during this type of exercise, sodium retention occurs despite increased ANP concentration. It seems therefore that short, intense exercise produces increases in ANP that resolve quickly during recovery. In contrast, lower intensity and very prolonged exercise causes ANP concentration to be significantly raised for a period of days following cessation of activity (Milledge et al. 1982). Figure 16.8Comparative percent changes of atrial natriuretic peptide (ANP) from pre-exercise in studies assessing ANP in healthy (Barletta et al. 1998; Schmidt et al. 1990; Somers et al. 1986) and diseased (Steele et al. 1997; Wisén et al. 2011) subjects. Dynamic incremental cycle tests (Barletta et al. 1998; Schmidt et al. 1990; Somers et al. 1986; Steele et al. 1997; Wisén et al. 2011) and an isometric handgrip test (Barletta et al. 1998). △ = maximal or end exercise, ⚬ = post exercise at five minutes (Barletta et al. 1998; no post measures for cycle test), six minutes (Steele et al. 1997), 10 minutes (Somers et al. 1986), 15 minutes (Schmidt et al. 1990), or 30 minutes (Wisén et al. 2011). CHF, chronic heart failure; MDD, major depressive disorder. Taken together, these studies clearly demonstrate the rise of ANP with exercise in healthy and unhealthy humans. Inconsistent results have been reported regarding the effects of exercise in hypoxia on ANP concentration. Schmidt et al. (1990) studied exercise while breathing air or reduced oxygen (PIO2 92 mmHg) and found that with both maximal and submaximal exercise the ANP response to exercise was reduced under hypoxic conditions. In contrast, Lawrence and Shenker (1991), using less severe hypoxia (PIO2 114 mmHg) and exercise at an intensity of 70–75% of maximum heart rate for 30 minutes, found that hypoxia enhanced the ANP response. A third study, using a decompression chamber to simulate an altitude of 3000 m (barometric pressure 520 mmHg, PIO2 99 mmHg) and a progressive exercise test to maximum, showed a reduced ANP response (Vuolteenaho et al. 1992). The reasons for these differing results are not apparent. Milledge et al. (1989) reported plasma ANP concentrations in 15 subjects before and after trekking from 3100 m to 4300 m and found no significant changes. Bärtsch et al. (1991b) measured plasma ANP concentration the morning after ascent on foot to 4559 m and found no changes in nine climbers who remained free of AMS but elevated levels in the five climbers who became ill. These subjects had a history of HAPE and were shown by echocardiography to have increased atrial diameters at altitude. In these cases, the increase in ANP concentration likely resulted from an increase in pulmonary artery pressure. Kawashima et al. (1992) showed that in HAPE-susceptible individuals, breathing an FIO2 of 0.10 resulted in a greater rise in ANP levels than in healthy controls, and that the rise correlated with the rise in pulmonary artery pressure. With more prolonged exposure to hypoxia, the effect of maximum exercise in raising ANP concentrations is reduced (Robach et al. 2000); however, maximal work rate (and maximal oxygen consumption) is also reduced at altitude. It is unclear therefore if the reductions in ANP concentration are due to this or due to some influence of acclimatization per se. Brain natriuretic peptide (BNP), also referred to as B-type natriuretic peptide, but has not been studied as extensively in hypoxia as ANP. Although first discovered in porcine brain, it is now understood to be mainly derived from heart muscle in humans (Hall 2005). It is released as pro-BNP from heart muscle in response to ventricular stretch (Goetze et al. 2003; Kinnunen et al. 1993; Magga et al. 1994) and possibly hypoxemia (Casals et al. 2009) and, once cleaved to its active form, exerts diuretic and natriuretic effects like ANP and reduces renin and aldosterone levels (Hall 2005), and possibly counteracts some of the hypoxia-induced pulmonary vasoconstriction (Cargill and Lipworth 1995; Ge et al. 2011). Acute hypoxemia significantly increases BNP and proBNP gene expression in human cardiomyocytes in vitro (Casals et al. 2009), in vivo (Goetze et al. 2003), and in porcine heart (Goetze et al. 2004). However, human in vivo hypoxia studies have reported contradictory findings. Toshner et al. (2008) and Feddersen et al. (2009) measured proBNP in subjects after ascent to 5200 m and 5050 m, respectively, and found no significant increase in proBNP or ANP, which Toshner et al. (2008) suggested was evidence that ventricular function is well-preserved at high altitude. Feddersen et al. (2009) also found increased nocturnal urine volumes following ascent, but these changes did not correlate with proBNP levels, indicating that BNP is not involved in altitude diuresis. Likewise, Woods et al. (2011b) found no significant rise in BNP even with an SpO2 of 68.1 ± 1.1% (±standard error of the mean) in hypobaric hypoxia equivalent to 5334 m. However, the same group (Woods et al. 2011a) found that BNP levels were often raised after trekking to 5150 m, especially in those with AMS. The same group reported a follow-up study of individuals trekking to 5643 m (Woods et al. 2012a) and showed that BNP and proBNP levels increased with altitude, and were still elevated after a nights rest and that those with the greater BNP response had significantly more AMS than those with less or no response. Pagé et al. (2015) found increased BNP following exercise at altitude. Concentrations were not increased at rest, however, and the highest concentrations were found in those subjects with the most severe AMS and highest pulmonary artery systolic pressures. Heinonen et al. (2014) found no increase in the N-terminal part of proBNP after 60 minutes of normobaric hypoxia (FIO2 0.11). Consistent with these observations, it has been reported that NT-proBNP is unchanged at altitude without exercise (Toshner et al. 2008). The studies that have found elevations in BNP have typically included prolonged trekking activity at high altitude (Pagé et al. 2015; Woods et al. 2011b; Woods et al. 2012a); therefore, it would seem that, in vivo, extended ventricular stretch is probably a greater stimulus to BNP secretion than hypoxemia. Adrenomedullin (AD) has many biological effects including dose-dependent increases in renal blood flow, urine output, and urinary sodium excretion (Hinson et al. 2000). It is expressed in many tissues throughout the body, including the lung, kidneys, and heart (Hofbauer et al. 2000). In particular, AD is released from endothelial cells in response to hypoxia via hypoxia-inducible factor (HIF)-1 (Cormier-Regard et al. 1998; Hofbauer et al. 2000). Toepfer et al. (1998) reported raised levels of plasma AD in subjects (all of whom had AMS) on the first and second days after arrival at 4559 m. Haditsch et al. (2007) measured plasma and urinary AD at multiple points during a trek to 5150 m and showed that urinary AD correlated significantly with nocturnal diuresis and sodium excretion, while plasma AD, although raised at altitude, did not. Their data suggest that renal AD is involved in the water and sodium diuresis of early altitude acclimatization. The effect of exercise on the AD response is unclear. Whether exercise increases or decreases circulating AD is dependent on several factors including sympathetic nervous activity, exercise type and duration, and RAA system status. It can be said, however, that AD, along with angiotensin II and endothelin I, contribute to the status of vascular tone throughout environmental and exercise challenges. This topic has been reviewed in detail elsewhere (Krzeminski 2016). On ascent to altitude, there is stimulation of the adrenal cortex by ACTH and cortisol is secreted. Early work documented this as a rise in 17-hydroxycorticosteroid (17-OHCS) during the first few days at altitude, which decreased to control values by days 5–7 (MacKinnon et al. 1963; Moncloa et al. 1965). This was later confirmed by Sutton et al. (1977) who showed that with the elevation of plasma cortisol there was a decrease in its normal diurnal variation on the first day of altitude exposure. Richalet et al. (1989) conducted a chamber experiment and found elevation of plasma cortisol with re-establishment of the diurnal variation after the first day at altitude. Following rapid ascents to 4300–5300 m, many of the subjects in these studies suffered from AMS, but even those free of symptoms showed this transitory rise in cortisol or its urinary metabolite. Over a more prolonged period at high altitude, early studies reported 17-OHCS levels were not significantly different from sea level values (Siri et al. 1969; Mordes et al. 1983). Woods et al. (2012b) measured morning and afternoon salivary cortisol in 47 trekkers traveling from Kathmandu (1300 m) to 5100 m on the trail to Everest base camp and found that this modest exercise resulted in a small rise in salivary cortisol at all altitudes. Interestingly, they found a fall in cortisol levels with increasing altitude in both pre-exercise (morning) and postexercise samples except at the highest point of 5100 m. They found a significant correlation of cortisol postexercise with arterial oxygen saturation, but no correlation with AMS scores (Woods et al. 2012b). Supporting this latter study, in a well-designed study with a comparatively large sample, (N = 34) a U-shaped cortisol response was found during ascent to 7050 m (von Wolff et al. 2018); the serum cortisol concentration dropped significantly on ascent to 4844 m then rose with progressive altitude before normalizing after seven days of acclimatization at 6022 m. Mean cortisol levels stayed within normal ranges throughout all altitudes. The mechanisms for this pattern of responses are unclear. Cortisol is sensitive to physiological stressors, so increases in sympathetic nervous activity seen at higher altitudes could be expected to stimulate cortisol secretion; however, it seems there is much intraindividual variability in cortisol responsiveness, and in general, cortisol seems to increase only at higher elevations. The role of exogenous corticosteroids, in particular dexamethasone, in the prevention and treatment of acute altitude illness is discussed in Chapters 20–22. The sympathoadrenal system is activated following ascent to high altitude with subsequent evolution in the response as the time spent at altitude increases. Acute hypoxia increases heart rate at rest and on exercise (Maher et al. 1975b), likely due to sympathetically mediated stimulation of β-adrenergic receptors on heart muscle cell membrane. Within four hours of arrival at altitude, stimulation of the adrenal medulla by hypoxemia causes an increase in arterial epinephrine (Mazzeo and Reeves 2003). Bouissou et al. (1989) also found a 32% increase in norepinephrine after 48 hours at altitude. Levels of epinephrine reach a peak on about days 2 to 4 and then decline as hypoxemia is relieved by increasing ventilation and hemoglobin concentration over the next seven to 14 days (Mazzeo and Reeves 2003). Berger et al. (2011) found that central venous and arterial epinephrine positively correlated with systolic blood pressure, heart rate, and pulmonary blood flow. Exercise results in a further rise in epinephrine depending on the work rate and degree of hypoxemia. Norepinephrine is released by sympathetic nerves and about 10–20% spills over into the circulation. In contrast to epinephrine, norepinephrine concentration rises only slowly, in line with increasing ventilation, reaching a plateau after 10–14 days at altitude. As with epinephrine, norepinephrine concentrations rise with exercise depending upon the workload but if workloads relative to V.O2,max are compared, the rise at altitude is similar to that seen at sea level (Mazzeo and Reeves 2003). Beyond the commonly cited effects on the systemic circulation, consideration has also been given to the effects of sympathetic activity on the pulmonary circulation. Duplain et al. (1999) demonstrated that HAPE-susceptible individuals have increased sympathetic activity, as assessed by postganglionic nerve discharge in skeletal muscle, compared to healthy controls, while other studies have shown that sympathetic stimulation via alpha receptors augments hypoxic pulmonary vasoconstriction (Brimioulle et al. 1997; Ming and Wang 1989), and administration of the alpha antagonist, phentolamine, reduces pulmonary vascular resistance and pulmonary artery pressure in individuals with HAPE and healthy controls at high altitude (Hackett et al. 1992). Berger et al. (2011), however, also found that the rise in pulmonary artery pressure and vascular resistance is largely independent of the sympathetic nervous system. This may be due to the opposing effects of vasodilation induced by β2-adrenergic receptor stimulation and vasoconstriction resulting from α1-adrenergic receptor activation. Extended stay at altitude induces chronically heightened and continuing catecholamine and sympathetic nervous activity, even while heart rate and cardiac output fall back toward sea-level values after several days at high altitude. During exercise, maximum heart rate is typically 140–150 beats min−1 at 5800 m compared with 180–200 beats min−1 at sea level (Chapter 18). This reduction in maximal heart rate and cardiac output takes place at a time when the plasma and urinary catecholamines are higher than at sea level. Cunningham et al. (1965), for example, reported elevated plasma and 24-hour urinary catecholamines during 17 days at 4559 m on Monte Rosa. There was no significant change in epinephrine but the increase in norepinephrine was greater on day 12 at altitude. Pace et al. (1964) found similar results at 3850 m, with urinary norepinephrine excretion rising slowly during 14 days at altitude without change in urinary epinephrine secretion. In Operation Everest II, at extreme altitude (PB ∼282 mmHg) after 40 days in the chamber, resting plasma norepinephrine was raised but epinephrine was reduced. On maximum exercise, likely owing to the concomitant reductions in maximal oxygen consumption, values for both catecholamines fell with increasing altitude (Young et al. 1989a). At least at rest, in subjects who had spent more than 10 weeks above 6000 m, the plasma norepinephrine concentration was found to be almost three times normal (Anand et al. 1993). Calbet (2003) studied nine subjects during a nine-week stay at 5260 m and found an increase in systemic blood pressure with elevations in plasma epinephrine and norepinephrine. Both systolic and diastolic pressures were significantly increased, likely reflecting heightened vascular tone and hypertension as a result of the increased adrenergic activity. Blood norepinephrine spillover from the leg was also increased compared with sea-level values. On the same expedition, Hansen and Sander (2003) measured sympathetic activity directly by peroneal microneurography after four weeks at altitude and found activity to be about three times sea-level values, suggesting that increased sympathetic activity persisted for many weeks after return to sea level. Bogaard et al. (2002) studied the effect of blocking either the sympathetic or parasympathetic arms of the autonomic system in a group of subjects at 3800 m on the heart rate and cardiac output on exercise. Propranolol had the expected effect of reducing heart rate, and glycopyrrolate increased the maximum heart rate to sea-level values. However, neither pharmacological blockade had any effect on maximum cardiac output, V.O2,max, or work rate at altitude, though, of course, these values were lower than sea level. Mazzeo et al. (2003) showed that blocking the α-adrenergic system in a group of female subjects at 4300 m resulted in increased norepinephrine levels compared with unblocked controls both at rest and with exercise. Women appear to have the same sympathoadrenal response to altitude as men with no differences attributable to the menstrual cycle (Mazzeo and Reeves 2003). Gosney et al. (1991) studied the adrenal and pituitary glands of five lifelong residents of La Paz who lived at 3600–3800 m and compared their glands with those of controls from sea level. The adrenal glands were significantly bigger, by about 50%. The pituitary glands were not larger but contained more corticotrophins. They surmised that greater amounts of ACTH were required to maintain adrenal function, perhaps because of hypoxic inhibition of adrenocortical sensitivity. However, in contrast, Ramirez et al. (1988) found no such inhibition. Sherpa high altitude residents do not suffer the same heart rate limitation on maximal exercise as noted above. Their heart rates can go up to 190–198 beats min−1 at 4880 m (Lahiri and Milledge, 1967). It is also noteworthy that directly measured sympathetic nerve activity is blunted in the Sherpa compared to lowlanders at 5000 m (Simpson et al. 2019). The endothelins are a family of peptides produced by a wide variety of cells affecting mainly blood vessels. Endothelin-1 (ET-1), clinically the most important member of the family, is the most potent vasoconstrictor yet discovered with about 100 times the activity of norepinephrine. ET-1 is produced by the endothelium and as much as 75% of the production is exported from the side of the cell opposite to the vessel lumen, where it acts on the adjacent smooth muscle without contributing to the plasma pool. In this way it perhaps should be considered as mainly a paracrine, rather than an endocrine, hormone. However, plasma levels probably do reflect the output of ET-1 and parallel the severity of the condition in, for instance, congestive cardiac failure (Holm 1997; Levin 1995). Other members of the family identified are ET-2 and ET-3, which are more localized to certain organs. All three peptides bind to the same two receptors, A and B, though with differing binding affinities. Synthetic inhibitors of these receptors, which are now used in clinical practice to treat pulmonary arterial hypertension, have helped elucidate some of the actions of these peptides. After Horio et al. (1991) showed that ET-1 increased in rats with increasing hypoxia, a number of studies in humans at altitude have shown that the rise in ET-1 is heavily influenced by the degree of exercise and hypoxia (Antezana et al. 1995; Boos et al. 2016; Cargill et al. 1995; Morganti et al. 1995). Blauw et al. (1995) studied the effect of acute hypoxia (SpO2 80%) and ET-1 infusion on forearm blood flow. Forearm blood flow was not changed by hypoxia, but plasma ET-1 concentration was increased. They concluded that hypoxia causes release of ET-1 from the pulmonary circulation but that this had no influence on peripheral vascular tone. Cruden et al. (1998) measured both ET-1 and big ET-1 in mountaineers ascending above 2500 m. A precursor peptide cleaved into ET-1, big ET-1 is a considerably less potent vasoconstrictor than ET-1, but exists in large enough quantities in plasma to produce high levels of ET-1. Both were increased on ascent to >2500 m, indicating that the increase in ET-1 was due to increased production and not decreased elimination. After three weeks at altitude, levels had returned to baseline values. Exercise had no effect on endothelin levels. In a separate study, they also found increases in both ET-1 and big ET-1 with cold exposure (Cruden et al. 1999). Berger et al. (2009) studied the changes in ET-1 concentrations across the pulmonary circulation in 34 subjects at low and high altitude (4559 m). While there was net clearance of ET-1 across the pulmonary circulation at low altitude, at high altitude levels pulmonary venous concentrations were higher than pulmonary arterial concentrations, indicative of net gain of endothelin with transpulmonary transport. Both the absolute concentration and the veno-arterial concentration difference correlated with the pulmonary artery systolic pressure. Droma et al. (1996b) reported detailed findings from a single case of HAPE with pulmonary hypertension and found ET-1 levels elevated on admission. The levels reverted to normal as the patient recovered and pulmonary artery pressure fell. The same team (Droma et al. 1996a) later demonstrated that HAPE-susceptible subjects had a greater hypoxic vascular response than controls but no significant change in ET-1 levels with a short hypoxic challenge (five minutes, FIO2 0.10). Sartori et al. (1999) studied HAPE-susceptible and HAPE-resistant mountaineers following ascent to 4559 m and demonstrated 33% higher ET-1 concentrations in the HAPE-susceptible individuals when compared to the controls as well as a significant direct relationship between changes in ET-1 concentration and pulmonary artery pressure from low to high altitude. While these studies suggest that differences in endothelin metabolism may affect the risk of HAPE, no studies have demonstrated that administration on ET-1 antagonists are useful in HAPE prevention. Modesti et al. (2006) administered bosentan in a randomized, double-blind manner to healthy individuals at sea level and following ascent to 4559 m and demonstrated reductions in pulmonary artery systolic pressure and slight improvements in arterial oxygen saturation. The study did not include HAPE-susceptible individuals, however, and, as a result, no conclusions can be drawn about its role in prophylaxis. As discussed further in Chapter 18, maximum exercise capacity is reduced at high altitude and part of this limitation may be related to hypoxia-induced increases in pulmonary vascular resistance and pulmonary artery pressure and subsequent effects on right ventricular function. A potential role for endothelin in the changes in exercise capacity is suggested by a study by Naeije et al. (2010) in which they administered the endothelin receptor antagonist, sitaxentan, in a randomized double-blind manner to healthy subjects in both normobaric and hypobaric hypoxia and noted reductions in pulmonary vascular resistance and a 30% improvement in maximum oxygen uptake following sitaxentan administration. Low birth weight is a feature of pregnancy at high altitude (Chapter 4), and data suggest that ET-1, as well as nitric oxide (NO) concentrations, may account, in part, for this observation. Julian et al. (2008) measured blood flow in uterine arteries (UA) as well as ET-1 and NO metabolites (NOx) in venous blood of mothers in Denver (1600 m) and in Leadville (3100 m) and found that birth weight correlated with UA blood flow in both groups. The higher the ratio of ET-1/NOx, the more UA vasoconstriction and the lower the blood flow to the uterus. In both groups, this ratio was correlated with birth weight. However, in the low altitude mothers, ET-1/NOx ratios were clustered at the low end of the range, while the high altitude mothers’ ratios were spread out to higher ratios, suggesting that increased ET-1 and/or decreased NO production resulted in lower uterine blood flows and smaller babies at altitude. In 24 individuals with chronic mountain sickness (CMS), Ge et al. (2011) found higher serum ET-1, BNP, and vascular endothelial growth factor (VEGF) concentrations in the CMS group compared to healthy controls, which were positively correlated with mean pulmonary arterial pressure. They also found lower serum endothelial nitric oxide synthase in the CMS group. High ET-1, BNP, and VEGF could be a result of chronic hypoxia, or a reactive compensation to high pulmonary arterial pressure caused by high altitude. These findings further support the notion that natriuretic peptides and vasoactive agents modulate adaptation to extended hypoxemia and may be useful markers of pathophysiological prognostic evaluation. The thyroid axis seems to be mildly upregulated at altitude, which may play a role in the increased basal metabolic rate often found at high altitude (Chapter 15). Surks (1966) found elevated levels of thyroxine-binding globulin (TBG) and free thyroxine (T4) in the first two weeks at altitude (4300 m), with a peak at nine days. Kotchen et al. (1973), in a three day chamber experiment (3650 m equivalent), found increases in free and bound T4 but no changes in thyroid-stimulating hormone (TSH), suggesting a shift of T4 from extravascular to intravascular compartments rather than increased pituitary activity. These results have been confirmed in a number of field studies (Rastogi et al. 1977; Stock et al. 1978b), which showed levels returning toward control in the third week at altitude. Sawhney and Malhotra (1991) studied both acclimatized lowlanders and high altitude natives and found levels of triiodothyronine (T3) and T4 to be higher than sea-level residents. T4 concentration in red cells was decreased at high altitude but there was no change in levels of reverse T3 (rT3), TBG, and T4 binding capacity of TBG and thyroxine-binding prealbumin. They also found no change in TSH. In l-thyroxine-treated men they still found a rise in T3 and T4, suggesting the rise to be independent of pituitary stimulation. Von Wolff et al. (2018) found no change in TSH upon ascent to 4844 m and 6022 m until 7050 m when TSH was significantly increased. T4 increased at each new altitude, in some individual cases rising above the normal range, then decreased slightly with seven days acclimatization at 4844 m. It was suggested that because T4 has a longer half-life than T3, and the TSH and T3 results were unclear, T4 is a better indicator of altitude adaptations and thyroid axis activation (Nepal et al. 2013). These findings are broadly consistent with earlier studies (Mordes et al. 1983; Stock et al. 1978a) and also indicate that the altitude effect tends to override the effect of weight loss due to calorie deficiency (Barnholt et al. 2006; Benso et al. 2007). In a more mechanistic study, Richalet et al. (2010) studied the effect of altitude (three to four days at the Observatoire Vallot, 4350 m) on the hormonal response to a number of hypothalamic factors including TSH. They found that the response to intravenously administered TSH was not significantly different from that at sea level. Verratti et al. (2017) found no changes in TSH concurrent with decreases in free T4 and T3 (also nonsignificant) in seven women at 5050 m after an 11 day ascent profile. The basal metabolic rate is elevated during the first two weeks at moderate altitude and correlates with the free T4 (Stock et al. 1978a). At higher altitudes (above 5500 m), it remains elevated for months (Gill and Pugh 1964), as does T4 (Mordes et al. 1983). Mordes et al. (1983) also found evidence of impaired conversion of T4 to T3 at 6300 m. Perhaps there is a change in the set point for the pituitary negative feedback system, resulting in higher levels of TSH. They also found that the response of the pituitary to an injection of thyrotropin-releasing hormone was enhanced at 6300 m compared with sea level. A similar finding has been reported by Ramirez et al. (1995) in resident highlanders at 2600 m. Historically, iodine deficiency was a significant problem in mountainous areas of the world including the Pyrenees, Alps, Andes, and Himalaya. This mainly related to geological factors causing iodine deficiency in the soil and, hence, in the diet. Because hypoxia stimulates thyroid function, iodine deficiency causes more exaggerated hyperplasia, which contributes to extremely high rates of goiter in high altitude regions. Following initiation of universal salt iodization in 1993, iodine deficiency is less of an issue in mountainous regions (Tamang et al. 2019a; Tamang et al. 2019b) and, as a result, the incidence of goiter and other disorders of iodine deficiency have decreased significantly (Ministry of Health and Population 2007). Glucose is integral to basal physiological processes and can be influenced by both altitude and activity. Reductions in oxygen tension can result in upregulation of catecholamines and activation of the adrenal cortex, which, in turn can affect insulin sensitivity, insulin secretion, and serum glucose concentrations (Figure 16.9 and Chapter 15). Figure 16.9Simplified illustration of the proposed pathways influencing glucose levels during acclimatization to high altitude. FFA, free fatty acids; TGs, triglycerides; GLUT4, glucose transporter 4. See text for details. On acute exposure to hypoxia, there is a rise in fasting blood glucose of about 1.7 mmol L−1, followed by a fall toward control values by the end of a week. The effect of prolonged high altitude on plasma insulin concentration is variable, studies having found both reductions or no change (Bailey et al. 2004; Blume and Pace 1967; Debevec et al. 2014; Riedl et al. 2012; Stock et al. 1978a) and elevations (Bosco et al. 2019; Siervo et al. 2014; Williams 1975; Young et al. 1989b). A recent meta-analysis (Matu et al. 2018) found a positive association between severity of hypoxic exposure and the magnitude of increases in insulin, and suggested that extreme altitude may account for the larger increases in insulin some studies have seen. Increases in insulin may be part of the altitude-induced stress response indicated by the concurrent rise in plasma catecholamine and cortisol levels. In subjects acclimatized to high altitude, fasting blood glucose was found to be lower than at sea level by some (Blume and Pace 1967; Stock et al. 1978a) but unchanged by others (Sawhney et al. 1986). Singh et al. (1974) found a persistently raised glucose concentration after 10 months at altitude. Together these data indicate the degree of variability of the effects of chronic hypoxia on both insulin and glucose changes in lowlanders, Bosco et al. (2019), Benso et al. (2007), Sawhney et al. (1986), Siervo et al. (2014), and Braun et al. (2001) reported increases in glucose and/or insulin, while Stock et al. (1978a), Debevec et al. (2014), and Bailey et al. (2004) all found decreases in both. This disparity between studies demonstrates the range of methods and ascent styles used, which may also account for some variability. For example, Braun et al. (2001) tested after 52 hours of hypobaric hypoxia; Debevec et al. (2014) tested after 10 days of normobaric hypoxia; Benso et al. (2007) tested after some time (details not reported) at 5200 m and immediately following an ascent of Everest without supplemental oxygen; Siervo et al. (2014) assessed a group of 24 at Everest base camp, eight of whom summited Everest; and Bosco et al. (2019) tested two days after descent to 1300 m following 22 days at 5050 m or higher and a trek of 450 km overall. Changes in blood insulin and glucose concentrations at high altitude are summarized in Figure 16.10. Figure 16.10
Introduction
Hormones Involved in Salt and Water Regulation
Antidiuretic hormone
Acute Hypoxia and ADH
Chronic Hypoxia and ADH
ADH Responses to Increases in Plasma Osmolality
Renin-angiotensin-aldosterone system
Exercise and the Renin-Angiotensin-Aldosterone System
Effect of Hypoxia and Exercise on the Aldosterone Response to Renin
Hemodynamic Effects of the Renin-Angiotensin-Aldosterone System
Atrial natriuretic peptide
ANP and Hypoxia
Exercise in Normoxia
Exercise in Hypoxia
Brain natriuretic peptide
Adrenomedullin
Corticosteroids
Sympathoadrenal System
Acute hypoxia
Chronic hypoxia
High altitude residence
Endothelin
Hypoxia and endothelin
Endothelin and HAPE
Endothelin and long-term altitude residents
Thyroid Function
Basal metabolic rate
Iodine deficiency and goiter
Blood Glucose Regulation
Glucose and insulin concentrations
Stay updated, free articles. Join our Telegram channel
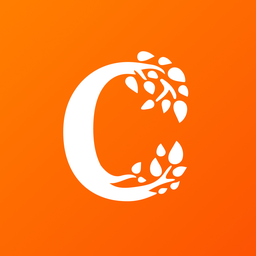
Full access? Get Clinical Tree
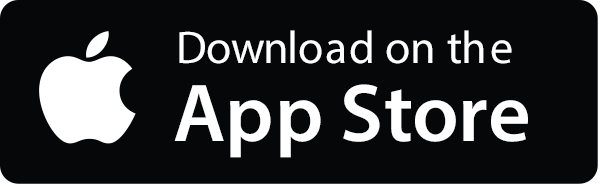
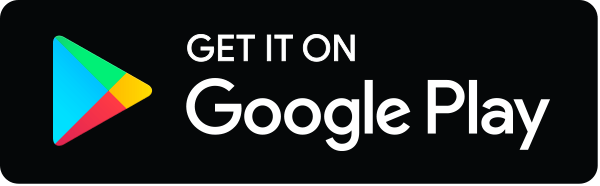