Coronary atherosclerotic disease is the leading cause of morbidity and mortality in the Western world. Atherosclerosis is a multifocal process generally confined to the intima of the coronary arteries. Despite advances in cardiovascular imaging, current diagnostic methods have been incapable of identifying coronary events prior to their occurrence. The goal of this chapter is to review the emerging invasive imaging technologies for the detection and characterization of high-risk atherosclerotic lesions prone to rupture and cause acute coronary syndromes. Although these newer technologies are in various stages of development, some with very limited human experience, preliminary results show promise and warrant our attention.
Prior to beginning a discussion of imaging modalities suitable for the assessment of the vulnerable plaque, some key questions need to be asked:
- 1
What is a vulnerable plaque?
- 2
What are the components of the vulnerable plaque that will be useful as targets for imaging?
- 3
What is the gold standard for identifying the vulnerable plaque?
- 4
Where are vulnerable plaques located in the coronary arterial tree?
- 5
How many vulnerable plaques can be present in a patient at one time?
- 6
What is the natural history of a vulnerable plaque?
- 7
Which patients will require screening?
- 8
What are the clinical implications of finding one or more vulnerable plaques?
- 9
What are the potential treatments, if any?
In an attempt to answer these questions, research has been extensive but many questions remain unanswered.
A culprit plaque or lesion is generally defined after a coronary event has occurred and is considered responsible for the coronary occlusion leading to the acute coronary syndrome. In contrast, an atherosclerotic plaque prone to thrombosis and susceptible to complications leading to a culprit lesion is described as a vulnerable plaque.
Retrospective histopathologic studies have identified several forms of vulnerable plaques. The most commonly occurring vulnerable plaques are thin-cap fibroatheromas (TCFAs), plaque erosion, and calcified nodules.
Rupture of a TCFA is the most common type of plaque complication and accounts for up to 65% of symptomatic thrombotic events. Characteristics of a TCFA lesion include the following: (1) a relatively large lipid core comprising more than 40% of the plaque volume; (2) a thin fibrous cap (<100 µm); (3) inflammation with infiltration of macrophage and lymphocytes at the cap shoulders; and (4) a paucity of smooth muscle cells. The second most common type of vulnerable plaque, responsible for approximately one third of acute coronary syndromes, is plaque erosion. These plaques are characterized by thrombus overlying de-endothelialized segments of vessel but with otherwise intact plaque. Five percent of thrombotic events have been ascribed to nodular calcifications protruding from the vessel wall with overlying thrombus. A less frequent type of vulnerable plaque, characterized by the overgrowth of the vasa vasorum, can lead to intraplaque hemorrhage and thrombosis.
Proximal and midportions of major coronary arteries have been shown to be the most frequent sites of plaque rupture. These proximal segments of coronary arteries are thought to harbor plaques with relatively larger lipid cores compared with plaques located more distally. Furthermore, two thirds or more of culprit plaques responsible for symptomatic thrombotic events are non–flow-limiting (<50%). , As a corollary, the angiographic diameter stenosis has not been shown to correlate with future thrombotic events.
A number of caveats need to be considered. According to autopsy, , angiographic, and intravascular ultrasound studies, patients presenting with an acute coronary syndrome may have documented plaque ruptures remote from the culprit lesion. Furthermore, plaque rupture is not synonymous with a clinically symptomatic thrombotic event and almost 5% to 15% of noncardiac deaths are found to have plaque ruptures in the coronary arterial tree. Recent reports have stressed the importance of thinking beyond the single vulnerable plaque and taking a more systemic approach (i.e., the vulnerable patient).
What is unknown at the moment is the natural history of the vulnerable plaques. Information about the evolution of vulnerable plaques is based on retrospective data obtained from histologic ex vivo and in vivo experiments. Imaging modalities capable of characterizing atherosclerotic lesions may prove helpful in understanding their natural history and detecting lesions that are at high risk for future cardiac events.
Several diagnostic imaging tools exist that are capable of evaluating determinants of plaque vulnerability. Certain high-risk features of the vulnerable plaque have been the target for vulnerable plaque imaging. Among the most common of these include the TCFA, lipid pool, and regions of high stress and inflammation. This chapter provides an overview of emerging techniques for the invasive imaging of the vulnerable plaques: (1) optical coherence tomography; (2) intravascular virtual histology; (3) intravascular palpography; (4) intravascular thermography; (5) intravascular magnetic resonance imaging; (6) Raman spectroscopy; and (7) near-infrared spectroscopy.
Optical Coherence Tomography
Optical coherence tomography (OCT) is a light-based imaging modality resulting in near-field intensity images of tissues with high resolution. In contrast to intravascular ultrasound imaging, OCT catheters include a fiberoptic core and use a superluminescent diode to emit a scanning beam of low-coherence, near-infrared light perpendicular to the catheter axis. Back-reflection of the emitted infrared light is then captured and, using interferometry to determine the penetration depth, an image can be reconstructed. Generally, to minimize energy absorption in the light beam by compounds found in blood, a center spectrum wavelength of approximately 1310 nm is used. OCT allows for in vivo real-time imaging at a resolution that is significantly higher (approximately 10 times) than that of intravascular ultrasound. The reported axial resolution of OCT is 10 to 15 µm and 20 to 25 µm for out of plane resolution. This improved resolution comes at the expense of poorer penetration through blood and tissue (1 to 3 mm). ,
Cardiovascular applications have until recently been limited to in vivo experiments using postmortem aortic and coronary artery specimens. , In the last few years, OCT has become increasingly popular to follow the evolution of the newer generation of intracoronary stents. , OCT can correctly characterize individual plaque components. Decreased signal areas with poorly delineated borders is typical of a lipid-rich pool, whereas a sharply delineated region with a signal-poor interior is characteristic of a fibrocalcific plaque. A fibrous plaque is clearly identified as a homogenous signal-rich lesion ( Fig. 17-1 ). Current OCT technology has demonstrated a sensitivity and specificity of 71% to 79% and 97% to 98% for fibrous plaques, 95% to 96% and 97% for fibrocalcific plaques, and 90% to 94% and 90% to 92% for lipid-rich plaques, respectively.

Although OCT correlates well with many of the features found on histologic sections, a recent study by Manfrini and colleagues called into question the ability of OCT to identify certain plaque features. After classifying 79 postmortem human coronary arterial sections by histology, OCT was able to identify 45% of fibrous cap atheromas correctly (κ = 0.27; P < .01), 68% of fibrocalcific plaques (κ = 0.40; P < .001), 83% of fibrous plaques (κ = 0.37; P < .001) and 100% of complicated lesions (all thrombi; κ = 1; P < .001). Because of the low OCT signal penetration, lipid pools or calcium behind thick fibrous caps were difficult to characterize.
In several studies, the variance of the OCT signal has demonstrated good correlation with the density of macrophages found in the fibrous cap of plaques on histologic section and thus may serve as a surrogate marker of plaque inflammation. OCT provides a more precise delineation of the thin fibrous cap, lipid pool, and fibrous plaque than gray-scale intravascular ultrasound. ,
In vivo studies have shown OCT to provide discriminatory information on plaque morphology and composition in patients presenting with coronary artery disease. In one study, patients who presented with an acute myocardial infarction were demonstrated to have lesions associated with thinner fibrous caps versus stable angina patients undergoing percutaneous coronary intervention (PCI) (median values [interquartile range], 47.0 µm [25.3 to 184.3 µm] vs. 102.6 um [22.0 to 291.1 µm] respectively; P = .02).
The ability of OCT to characterize atherosclerotic plaques is limited by its low depth of penetration (1 to 3 mm) and therefore full circumferential views of larger coronary arteries (>3.5 mm) may not be possible. Furthermore, signal scattering and signal attenuation caused by blood requires that blood be displaced by contrast agent flushing or low-pressure occlusive balloon inflations, resulting in a shortened time window for imaging (∼2 seconds). Newer generation rapid-scan OCT systems are being developed to reduce blood-free imaging times. These newer systems use a wider range of light wavelengths (1200 to 1360 nm) and then measure the back-reflected echo time. Using Fourier transformation techniques to convert frequency domains to time domain representations, these newer systems permit image acquisitions four to five times faster. Long arterial segments being scanned during a shortened low-pressure balloon occlusion or one-bolus contrast flush without occlusion.
Intravascular Ultrasound–Virtual Histology
Although gray-scale intravascular ultrasound is the current gold standard to assess coronary plaques and vessel dimensions, it cannot reliably differentiate among various tissue components of the atherosclerotic plaque. Intravascular ultrasound gray-scale images are formed by processing the acoustic reflectance of tissue. However, a substantial amount of information within and between the peaks of the radiofrequency signal is unprocessed. Further analysis of this radiofrequency data from the unprocessed backscattered ultrasound signal provides an alternative to the commonly used intravascular ultrasound gray-scale image analysis. By subjecting the radiofrequency data to different mathematical algorithms, various geometric reconstructions revealing important histologic information can be obtained. This section will focus on intravascular ultrasound-virtual histology (IVUS-VH).
IVUS-VH allows categorization of the atherosclerotic plaque into four distinct tissue components to create a colored tissue map: calcified plaque (white), fibrous plaque consisting of densely packed collagen (green), fibrolipid plaque consisting of collagen and interspersed lipid (greenish yellow), and necrotic core, including cholesterol clefts, foam cells, and microcalcifications (red; Fig. 17-2 ).


The accuracy of IVUS-VH was evaluated in 15 patients with stable angina and in 15 patients with acute coronary syndromes using in vitro histopathology specimens obtained by directional coronary atherectomy. The predictive accuracy of IVUS-VH was found to be 87.1% for fibrous, 87.1% for fibrofatty, 88.3% for necrotic core, and 96.5% for dense calcium plaques. Interestingly, IVUS-VH correctly identified a higher frequency of necrotic core in patients with non–ST-segment elevation myocardial infarction (NSTEMI) or unstable angina than in patients with stable coronary artery disease. However, directional atherectomy can disrupt the structural integrity of the tissue specimens; studies using it for comparison have therefore been criticized. In an atherosclerotic porcine model, Granada and associates compared 60 lesions evaluated by in vivo IVUS-VH to sections of histology. Unlike the previous study reported, the predictive accuracy of IVUS-VH was a disappointing 38% to 58%.
Using IVUS-VH, Rodriguez-Granillo and colleagues recently investigated the prevalence of TCFA and its relationship with clinical presentation. IVUS-derived TCFA was defined as a lesion fulfilling the following characteristics: (1) focal, rich necrotic core plaque (10% of circumferential surface area) in contact with the vessel lumen; and (2) atheroma volume of 40%. In this study, 23 patients with an acute coronary syndrome had a threefold higher prevalence of IVUS-derived TCFA versus 32 stable angina patients ( P = .018). There was no association between patient baseline characteristics and the presence of IVUS-derived TCFA. Furthermore, 66.7% of all IVUS-derived TCFAs were clustered within the first 20 mm.
Other studies have assessed the relationship between plaque composition and vascular remodeling and clinical presentation with IVUS-VH. Areas of positive remodeling have been associated with a relatively higher content of lipid necrotic core and lower amounts of fibrous tissue. , Furthermore, coronary plaques of patients presenting with acute coronary syndrome were found to have an increased percentage of lipid core, whereas those of stable angina patients were found to have an increased fibrous content. No difference was noticed in mean calcium or fibrolipid percentage between the two groups ( Fig. 17-3 ).

The reproducibility of vessel geometry and plaque composition using IVUS-VH was examined in 16 nonintervened lesions during elective PCI. There were small relative interobserver differences (<5%) for geometric measurements of vessel diameter and cross-sectional area. In contrast, differences were more notable for calcium cross-sectional area and necrotic core (<10%), and even more notable for fibrous (10%) and fibrolipid (24%) cross-sectional area.
The threshold value of 65 µm for defining a thin fibrous cap originated from postmortem studies. Significant shrinkage of the collagen fibers of up to 80% can occur during the postmortem fixation process and, therefore, a TCFA may be larger than 65 µm. On a practical level, the axial resolution of IVUS-VH is approximately 246 µm; therefore, the absence of visible fibrous tissue overlying a necrotic core indirectly suggests a cap thickness of less than 246 µm and the absence of such tissue by IVUS-VH is used to define a thin fibrous cap. ,
The current limitation of IVUS-VH is the relatively long amount of postacquisition processing required to analyze a region of interest accurately. The potential application of IVUS-VH to identify high-risk atherosclerotic lesions and predict adverse coronary events is currently undergoing clinical evaluation in an international, multicenter, industry-sponsored prospective trial (PROSPECT trial).
Palpography
Atherosclerotic plaques can have different mechanical stress properties, depending on their compositional tissue characteristics. Plaque rupture has been known to occur in areas with increased mechanical stress. Stress on a plaque can increase because of the following: (1) caps become thinner; (2) lipid pools become larger; (3) difference in stiffness between the cap and lipid pool increases; and (4) inflammation weakens the cap. , In vivo, stress is imparted on the vessel lumen by the pulsatile action of intravascular blood pressure, which strains the vessel wall. At a given pressure difference, soft tissues (e.g., lipids) will deform more than hard tissues (e.g., calcium). , The rate of deformation (i.e., strain) of the tissue is directly related to the mechanical properties of the tissue being investigated. Palpography assesses the first 450-µm layer of the plaque and allows for the measurement of radial stress by obtaining one strain value per angle at the lumen vessel. Because palpography only requires ultrasound data sets that are acquired at different levels of intraluminal pressure, it can be realized using conventional, clinically used catheters. IVUS palpography images are constructed using the relative local displacement between IVUS images obtained during two different intravascular blood pressure recordings and plotted as a color-coded contour at the lumen vessel boundary. Regions of low strain are labeled blue and regions of high strain are labeled red to yellow. A complementary image is obtained by combining the color-coded strain information and the IVUS image. With reference to IVUS palpography, a vulnerable plaque is defined as a plaque with a high strain region present at the lumen boundary surrounded by low strain values.
The Rotterdam classification (ROC) score uses a 1 to 4 grading scheme to categorize plaque strain values based on the degree of tissue in proportion to the applied pressure: ROC I, 0% to <0.6%; ROC II, 0.6 to <0.9%; ROC III, 0.9 to <1.2%; and ROC IV, 1.2%. A high strain spot is defined by strain values more than 1.2% (at 4-mm Hg pressure difference) that spans an arc of at least 12 degrees at the plaque surface adjacent to an area of low strain less than 0.5% (at 4-mm Hg pressure difference). ,
As opposed to gray-scale IVUS images, IVUS palpography can potentially differentiate between fibrous, fibrofatty, and fatty components of plaques based on inherent differences in strain values. Animal studies using Yucatan pig models have demonstrated fatty plaques to be associated with higher mean strain values and high strain spots to correlate with macrophage concentration. Using postmortem histologic sections of coronary arteries, IVUS palpography was determined to have a sensitivity and specificity of 88% and 89%, respectively, for the identification of a vulnerable plaque. Furthermore, there was a correlation between higher strain levels and the amount of macrophages in the cap. Reconstructed three-dimensional palpograms from 55 coronary artery disease patients revealed that patients with stable angina had fewer deformable plaques per vessel (0.6 ± 0.6) than unstable angina (1.6 ± 0.7) or acute myocardial infarction (2.0 ± 0.7) patients. Furthermore, there was a positive correlation between C-reactive protein (CRP) levels and the number of deformable plaques (r 2 = 0.65; P < .001).
Thermography
The rich cellular environment of atherosclerotic plaques contributes to the generation of heat. Cells of the immune system produce endogenous pyrogens known as cytokines. These structurally diverse proteins are mediators of inflammation, angiogenesis, and numerous internal cellular processes. In addition to contributing to the generation of heat, cytokines mediate the acute-phase response by increased production of CRP, serum amyloid A, fibrinogen, complement proteins B, C3, and C4, interleukin-6, and a variety of proteinase inhibitors. Some of them, like tumor necrosis factor-α (TNF-α) and interleukin-1a, are able to increase endothelial cell adhesiveness and associated procoagulant effects. ,
Macrophages and, to a lesser extent, T and B lymphocytes are the main cytokine producers in atherosclerotic plaques. Macrophages are abundant in atherosclerotic plaques within the plaque, often observed in the shoulder region where the fibrous cap meets normal intima. , Dense cellular regions in specimens of carotid plaques showed significantly higher temperatures when compared with the neighboring fatty core, which is often densely populated by lipid-laden macrophages.
Another contributor to local heat production is plaque angiogenesis. Mediated by cytokines such as vascular endothelial growth factor, new capillaries that penetrate into the atherosclerotic plaque contribute to the elevation in the temperature profile.
There is a correlation between plaque angiogenesis and inflammation ; in addition, both are thought to be risk factors for plaque rupture. The release of matrix-digesting enzymes such as metalloproteinases secreted by macrophages soften the fibrous cap, enhancing the vulnerability of atherosclerotic plaques to rupture.
Because inflamed plaques are at high risk for rupture with subsequent thrombosis, the development of a catheter-based or noninvasive imaging technique able to detect and distinguish vulnerable plaques could be a valuable tool for assessing prognosis and developing treatment strategies. Oscillations in the temperature of an inflamed or heat-vulnerable plaque have led to a new technique that attempts to detect local temperature fluctuations of different plaques.
It is now clear that before a plaque rupture, vulnerable plaques experience active inflammation and progressive matrix degeneration. Despite improvements in coronary imaging and identification of inflammatory markers, plaque rupture cannot be predicted by clinical means. Casscells and coworkers were the first to postulate that plaques covering areas infiltrated by monocytes and inflammatory cells could be identified by the heat generated and released by the activated inflammatory cells. Further studies have shown that ex vivo atherosclerotic plaques taken during carotid endarterectomy have thermal heterogeneity: Plaques with dense macrophage infiltration give off more heat than noninflamed plaques, and plaque temperature varies inversely with the thickness of the fibrous overlying cap. With the later development of a catheter-based technique to measure the temperature of human coronary arteries, thermal heterogeneity within human atherosclerotic coronary arteries was demonstrated. This heterogeneity is markedly greater in patients presenting with unstable angina or acute myocardial infarction, implying that this heterogeneity may be related to the pathogenesis of these syndromes. Furthermore, the presence of higher temperatures at the site of culprit lesions in patients with unstable angina or myocardial infarction is associated with higher levels of CRP and serum amyloid A.
An experimental rabbit atherosclerotic model demonstrated a direct correlation between macrophage content in atherosclerotic plaques and temperature variations. In addition, lipid-lowering therapy not only reduced macrophage plaque content, as determined by histology, but subsequently resulted in reduced temperature heterogeneity within the plaque. This suggests that thermography may be a novel method of following the macrophage burden of atherosclerotic plaques.
One of the few prospective studies to correlate plaque temperature and clinical outcomes involved 86 patients with stable coronary artery disease, unstable angina, and recent acute myocardial infarction ( Fig. 17-4 ). The difference in temperature between the atherosclerotic plaque and healthy vessel wall was a strong predictor of event-free survival 1.5 years after a PCI in patients with coronary artery disease. The mean change in temperature was greater in patients with adverse cardiac events within each subgroup, although there was a nonsignificant trend in patients with a recent acute myocardial infarction. The threshold value of temperature variation above which the rate of adverse cardiac events was significantly increased was 0.5%. Most of the cardiac events were related to restenosis of the treated lesion and not to a recurrent acute coronary syndrome.

Thermography has permitted some interesting observations on the nature of coronary plaques. In one small study involving patients with stable and unstable coronary artery disease, approximately one third of the lesions were found to be hot, indicating that plaque inflammation was fairly prevalent. In addition, the study found cold lesions in patients with unstable angina and hot lesions in patients with stable angina, suggesting that clinical presentation does not necessarily predict plaque morphology and temperature values. It was observed that patients with unstable coronary syndromes may have both hot and cold lesions only millimeters apart in contrast to findings suggesting diffuse vessel inflammation in patients with unstable coronary syndromes.
The potential confounding effects of variations in coronary blood flow on measurements of plaque temperature have been examined. , This “cooling effect” of coronary blood flow is thought possibly to underestimate the true temperature of the atherosclerotic plaque. Some have suggested that correct interpretation of intravascular thermography data requires additional complementary information, such as coronary blood flow and structural characteristics of the atherosclerotic plaques. A more recent prototype catheter enables temperature assessment during complete occlusion of the coronary blood flow and full apposition of the sensing elements.
Current limitations to the use of thermography in clinical practice include the following: (1) cutoffs must be determined for defining vulnerability; (2) accurate temperature assessment requires direct contact of the thermistors with the vessel wall and the potential, therefore, of endothelial damage; and (3) temperature changes can occur with coronary injections of fluids or contrast and thus are to be avoided before and during the measurements.
At present, strong pathophysiologic data support the role of heat production by vulnerable plaques. The technology to measure the heat within the coronary plaques precisely is available. However promising this technology is, larger clinical trials are required to determine the sensitivity and specificity before it becomes widely available.
Intravascular Magnetic Resonance Imaging
Magnetic resonance imaging is a nonionizing imaging modality that applies a surface magnetic field to biologic tissues. This results in a net alignment of the proton spins within the body parallel to the direction of the magnetic field. As short radiofrequency energy is applied, the protons absorb energy, and subsequently release it as the excited protons conform to their original state. These signals are detected by the receiver coils and subsequently interpreted.
Three imaging contrasts are used to determine plaque composition: (1) T1-weighted imaging; (2) T2-weighted imaging; and (3) proton density–weighted imaging. Information obtained about the rate of energy release (spin-lattice relaxation time) and the rate at which the spins diphase (spin-spin relaxation time) are referred to as T1- and T2-weighted measurements, respectively. Furthermore, proton density–weighted imaging can be obtained by adjusting the imaging parameters to reduce T1 and T2 contributions, leaving only the differences in water or lipid proton density.
The use of in vitro conventional magnetic resonance imaging techniques has been evaluated for the study of atherosclerotic plaques in human carotid arteries, , aorta, peripheral arteries, and coronary arteries. The carotid arteries, because of their relatively superficial location and immobility, provide the ideal setting for in vivo imaging of the atherosclerotic vessel by conventional magnetic resonance imaging.
In contrast to conventional magnetic resonance imaging of the carotid arteries, preliminary studies in an animal pig model have quickly demonstrated the limitations of assessing the coronary arteries by conventional magnetic resonance imaging in vivo. The most important limitations included cardiac and respiratory motion artifacts and the deeply situated and relatively smaller and nonlinear course of the coronary arteries.
To overcome the potential shortcomings of conventional surface magnetic resonance imaging, placement of an intravascular receiver coil in combination with an external magnetic field is feasible. The proximity of the magnetic resonance detector coil to the arterial wall would theoretically provide enhanced image quality. Intravascular coils have demonstrated good correlation between histologic sections and intravascular magnetic resonance imaging of human thoracic aortic segments. An important safety concern is vessel heating and injury caused by the energy emitted by the intravascular coil. Although the coronary blood flow may partially dissipate the heat, smaller vessels may be more prone to temperature increases.
More recently, Larose and colleagues have examined the use of intravascular magnetic resonance imaging using an intravascular detector coil and external magnetic field to characterize the structure of iliac arteries of 25 human subjects and compared the results with IVUS. They found that intravascular magnetic resonance imaging visualizes the inner and outer plaque boundaries, even in the presence of extensive calcification, a factor that precluded IVUS interpretation of the vessel wall.
Conventional magnetic resonance imaging, with or without intravascular coils, requires the application of external magnets and therefore a magnetic suite. It has made such evaluations impractical in the cardiac catheterization laboratory.
Topspin Medical (Wilmington, DE) has recently developed a self-contained intravascular magnetic resonance imaging probe to image the arterial wall without the need for the application of external magnets or coils. The intravascular magnetic resonance imaging probe exploits the concept of the apparent water diffusion coefficient. The self-diffusion of water molecules in a measured volume quantified by magnetic resonance imaging is referred to as the apparent diffusion coefficient (ADC) value. Previous studies using conventional magnetic resonance imaging have demonstrated that lipid-rich tissue has a significantly lower ADC value compared with that of fibrous tissue. However, the intravascular magnetic resonance imaging probe is not able to detect calcification deposits because of the relatively low concentration of hydrogen atoms available for excitation. In addition, the intravascular magnetic resonance imaging probe cannot differentiate between the fibrous cap and normal medial layer because both tissues have similar biophysical properties and, therefore, similar ADC coefficients.
The intravascular magnetic resonance imaging probe contains a strong static magnetic field gradient (∼300 T/m), which makes it more than suitable for diffusion-weighted imaging to measure ADC. The depth of penetration of the field of view is roughly 50 to 200 µm into the vessel wall. It is important to realize that the intravascular magnetic resonance imaging probe does not generate an actual morphologic reconstruction of the vessel wall, but displays a color-coded map indicating the lipid composition in the field of view of probe. The system is comprised of a console similar to an IVUS console, a catheter interface unit, and the catheter. The system can be used in a conventional cardiac catheterization laboratory. Current design technology uses a self-contained 5.2-Fr intravascular magnetic resonance imaging catheter and a conventional 300-cm angioplasty wire to advance the intravascular magnetic resonance imaging probe to a maximum distance of 5 cm from the orifice of the vessel.
The Topspin Medical intravascular magnetic resonance imaging probe was examined ex vivo using human aortic and coronary samples and then correlated to histologic and immunohistochemical analyses of the tissues. The probe correctly correlated with the histologic diagnosis in 15 of 16 (94%) aortic lesions and in 16 of 18 (88%) coronary lesions.
A first-in-man safety and feasibility study using the Topspin Medical probe has been completed in 29 patients. No major adverse cardiac events or device-related complications were observed. Lesions were classified as fibrous in 4, intermediate in 4, and lipid rich in 8. Six patients were excluded from analysis because of poor-quality images due to artifacts.
Further studies will be needed to validate the system for clinical use. Molecular imaging of atherosclerosis coupled with magnetic resonance imaging may provide alternative and more promising modalities for imaging the vulnerable plaque.
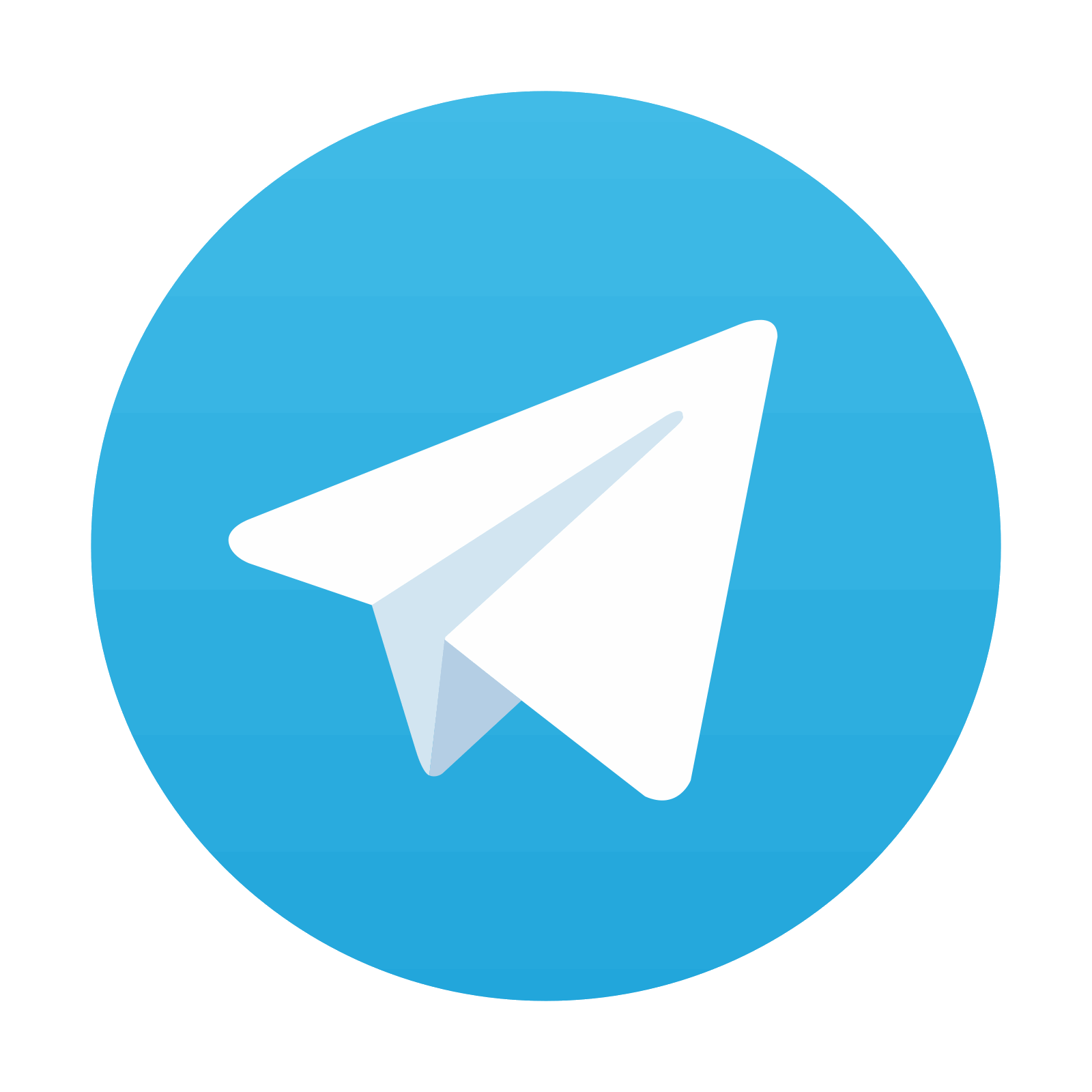
Stay updated, free articles. Join our Telegram channel
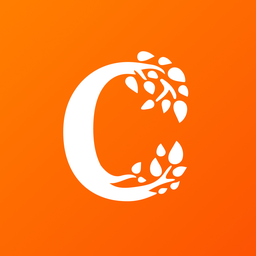
Full access? Get Clinical Tree
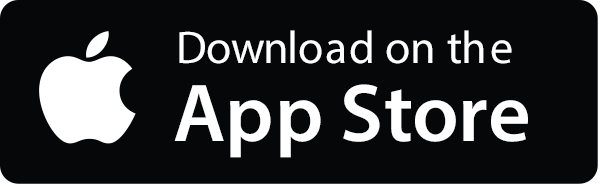
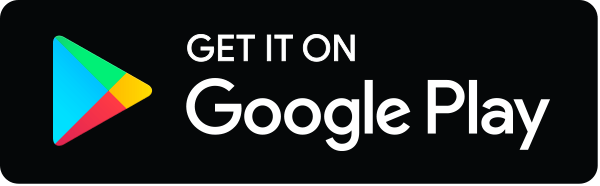