Embryology of the Lungs
Mala R. Chinoy
The field of embryology has contributed critical knowledge to scientific and medical investigations as well as having led to breakthroughs. The history of pulmonary embryology is no exception. As with other areas of human embryology, the first theories regarding lung development were derived from animal models, beginning with early air-breathing animal species and later work focusing on mammals and primates.
Several developmental principles and timelines elucidated by animal embryologists from early 1900s were directly applied to human lung development. Most enhanced our understanding of human lung embryogenesis, but others were not applicable to the human model and stalled advancement in the field.
As the work progressed, several investigators provided the nomenclature and systematic documentation of the developmental stages of human pulmonary embryology, thus contributing to a better understanding of pulmonary organogenesis. Concurrently, the scientists at Carnegie Institute during the first half of the 20th century were able to systematically document pulmonary organogenesis in relation to the development of the fetus as a whole. Over the next 20 years, major contributions specific to pulmonary development were made with reference to lung differentiation through airway and vascular morphogenesis. This eventually led to the structural and functional relationships of the developing lung. These contributions have been pivotal to our understanding of both pediatric and adult lung function and pathology, and continuing work on pulmonary development will yield many more meaningful strategies for surgical, medical, and genetic therapeutic approaches to pulmonary diseases.
This chapter focuses on prenatal pulmonary organogenesis, which is important to the understanding of the pulmonary morphogenesis and differentiation that continue after birth. Significant alveolar remodeling, growth, differentiation, and lung development occur from embryonic development to childhood, where it may extend up to 8 years or beyond in humans.
In this chapter, significant events are marked using crown–rump length and Carnegie stage, after Streeter.91,92,93,94 All dates given are timed from ovulation unless otherwise specified.
Lung development has two fundamental stages: the initial stage of establishment of the air-conducting system and the later stage of development of the respiratory surface area for breathing. Organogenesis of lung involves intricate interactions of two germ layers—endoderm and mesoderm—along with a host of growth factors, hormones, and complex signaling mechanisms. The mammalian lung develops by outpouching from the foregut endoderm as two lung buds into the surrounding splanchnic mesenchyme. Several different regions of the foregut are specified to develop into different thoracic and visceral organs. The lung buds further elongate and branch, and the foregut is separated longitudinally into esophagus and trachea. In mice and rats or rodents, this occurs around embryonic day 11, where the right lung bud develops into four different lobes and the left lung develops as a single lobe. In humans, these processes occur by 3 to 4 weeks of embryonic development (Fig. 2-1), where the right lung is a trilobar lung and the left lung is a bilobar lung. Several generations of dichotomous branching occur during embryonic development, followed by sacularization and alveolarization pre- and postnatally, transforming a fluid-filled lung into an air-breathing lung able to sustain the newborn. This transformation occurs through multiple phases or periods of morphogenic, structural, and functional transformation. During this development from the embryonic to the newborn stage, the lung’s architecture undergoes profound changes, which are marked by a series of programmed events regulated by master genes (e.g., homeobox genes), nuclear transcription factors, hormones, growth factors, and other factors. These programmed events can be altered by undesirable exposure to overdoses of hormones, vitamins, growth factors, synthetic drugs, environmental toxins, radiation, and other agents. In recent years molecular techniques have opened new avenues to the study of specific functions of genes or their products (proteins) in vivo or in vitro at the cellular and organelle levels. Some of these techniques include targeted disruption, knockin/knockout genes, in vitro mutagenesis, and the use of sense and antisense oligonucleotides.
Lung Formation
The regulation of lung organogenesis and branching morphogenesis leading to defined lung architecture, growth pattern, and differentiation involves cross-talk between epithelium, mesenchyme, and endothelium. Lung development is a tightly regulated process involving cross-regulation of the stimulatory and inhibitory genes in progenitor cells or stem cells in the lung. An understanding of these processes also provides a foundation for understanding the possible pathways involved in lung injury and repair.
A Brief Overview of the Developmental Timetable of the Lung
Lung development begins by the end of week 3 or early in week 4.
The respiratory epithelium develops from the germ layer, or endoderm.
The connective tissue, cartilage, and muscle are derived from splanchnic mesoderm and neural crest cells.
Respiratory Diverticulum
At 26 days (embryonic week 4), a small protrusion in the foregut appears.
It outpouches or evaginates to form a laryngotracheal diverticulum (Fig. 2-1).
Separation of the Esophagotracheal Ridge
Development of the esophagotracheal ridge begins as folds, followed by a longitudinal esophagotracheal septum (Fig. 2.2).
Development of the Larynx
Epithelium is derived from the endoderm of the laryngotracheal tube.
Mesoderm is derived from splanchnic mesoderm.
Cartilage is derived from neural crest cells.
Development of the Laryngeal Aditus
Laryngotracheal groove
Primitive glottis to laryngeal aditus
Development of the Lungs
The lung-bud outpouching develops by the late third or early fourth week of embryonic development.
It divides into two lung buds:
The right one develops into three main bronchi.
The left one develops into two main bronchi.
Bronchi continue to divide (Fig. 2-3).
By 6 months, there have been 17 generations of subdivisions.
Six additional divisions of the bronchial tree occur after birth.
With progressive lung growth, the lung parenchyma/distal lung grows and differentiates.
At birth the tracheal bifurcation is at the level of the fourth thoracic vertebra.
Developmental Stages and Structure of the Lung
During lung development, growth, and differentiation, there are four distinct structural stages, each with a characteristic developmental feature.* These stages are (a) the pseudoglandular stage, development of the bronchial tree; (b) the canalicular stage, development of acini and vascularization; (c) the saccular stage, further differentiation of the acini into saccules, increase in saccules and vascularization, as well as differentiation of the epithelial cells into type I and II pneumocytes; and (d) the alveolar stage, increase in the number of alveoli and extensive increase in surface area. In mice and rats, the process of alveolarization mainly occurs postnatally, whereas in humans it is a prenatal as well as a postnatal process (Table 2-1; Fig. 2-4).
As early as 1936, the initial classification of pulmonary developmental stages was provided by Dubreuil et al.38 Thurlbeck,98 a well-recognized pulmonary developmental biologist, provided a further defined classification of the stages of lung development in 1988. His stages were divided as follows: embryonic development of the lung occurs up to a week short of 3 months, the pseudoglandular stage comprises between 3 and 4 months (weeks 12–16), the saccular stage occurs between 4 and 7 months (weeks 17–28), and the alveolar stage occurs from 7 to 9 months, or to term (weeks 29–36). Table 2-1, as presented by O’Rahilly and Muller,74 depicts the variability suggested by different embryologists, where some of the stages appear to be overlapping. However, the stages defined by Thurlbeck98 are most widely accepted by scientific and medical communities.
Table 2-2 provides a comparison of the developmental stages of the lung in humans versus that in rodents commonly used as experimental animals in the study of lung organogenesis and the time periods for specific structural development of the lung.
Distinct Developmental Features of the Five Stages of Lung Maturation
Embryonic Period: Weeks 0 to 12
Smith86 first identified the formation of the esophagotracheal ridge in the foregut of a 3-mm Carnegie stage 10 fetus. This development, which begins in the late third or early fourth week, is summarized in Figure 2-1. At this point, the cranial ventral foregut has enlarged and an invaginating ridge of endodermal tissue has formed the esophagotracheal folds. These folds meet in the midline, defining a dorsal esophageal lumen and a ventral laryngotracheal primordia. Fusion of the esophagotracheal folds then progresses from caudad to cephalad. Subsequent necrosis and degeneration of intervening tissue allows separation of the esophagus and trachea. Both separated tracheal and esophageal primordia elongate. Complete separation is achieved only by day 41 (stage 17, 11–14 mm). According to Zackary and Emery,110 incomplete separation at this phase is the precursor for esophagotracheal fistulas and their associated anomalies.
The primordial lung bud develops from the distal end of the newly formed trachea. O’Rahilly and Boyden73 first visualized the lung bud in a day-26 fetus (stage 12, 4 mm), marking the beginning of the embryonic phase of lung development. The embryonic phase, which spans postovulatory days 26 to 52, focuses on the morphogenesis of the proximal airways and the early differentiation of adjacent mesenchymal tissue.
Shortly after lung bud is formed, right and left lung primordia are seen. As the embryonic mediastinum develops, the left lung main bronchus assumes a shorter and more horizontal orientation. This asymmetry appears to be independent of mechanical influences from the developing heart, which will not occupy its final position in the mediastinum until the seventh week. Axial growth, as well as dichotomous division of the airways, continues during the embryonic phase (Fig. 2-2). By 52 days after ovulation (stage 22, 23–28 mm), secondary bronchi are visualized, marking the end of the embryonic phase and the beginning of the pseudoglandular phase of development.
Pseudoglandular Period: Weeks 5 to 16
At this stage all elements of the lungs are developed except those involved in gas exchange; that is, the epithelial cells are
glycogen-laden and do not have surfactant storage bodies—the lamellar bodies—nor is there any surfactant secretion in what will become the airspaces. Therefore, fetuses born up to 16 weeks will not survive.
glycogen-laden and do not have surfactant storage bodies—the lamellar bodies—nor is there any surfactant secretion in what will become the airspaces. Therefore, fetuses born up to 16 weeks will not survive.
Table 2-1 Human Pulmonary Developmental Stages as Defined by Different Embryologists | ||||||||
---|---|---|---|---|---|---|---|---|
|
As the pulmonary primordial development proceeds from the embryonic to the pseudoglandular period, the bronchi continue to grow and mature. Histologically, the bronchi are lined with a glycogen-laden, cuboidal epithelium and the lung has a glandular appearance. The period during which this characteristic appearance of the lung is present spans weeks 5 through 16 of pulmonary embryogenesis and is termed the pseudoglandular period (or pseudoglandular phase, as suggested by Boyden11). During the embryonic period, development of the trachea and lung bud occurs. In the pseudoglandular period, there is proliferation of the endothelial, mesenchymal, and surrounding structures, including the conducting airways, the pulmonary vasculature, and the diaphragm.
During the embryonic period of development, poorly differentiated mesenchyme derived from the splanchnic mesoderm envelops the pulmonary primordia. The developing lung is dependent on the interaction/cross-talk with this mesenchyme. These interactions are critical all throughout the lung development as well as at later stages. Several investigators have shown organ-specific effects of mesenchyme through elegant experiments. Studies by Rudnick82 and Taderera96 have shown that both bronchial branching and pulmonary endothelial development are defective, abnormal, or inhibited in the absence of species-specific mesenchyme. As lung development progresses, this mesenchyme differentiates into vascular and other stromal connective tissue elements.
Early in the pseudoglandular period, vascular plexuses arising from the mesenchyme are seen forming around the lung bud. By the end of the pseudoglandular phase, vascular morphogenesis is well established and connections to the developing heart are formed.
Late in the pseudoglandular period, bronchial support structures are seen. A ringed cartilaginous exoskeleton was visualized by Bucher and Reid21 at the level of the trachea by week 10 and around segmental bronchi by week 16. The bronchi are not only elongating, but also branching throughout this period. In the right chest, the right mainstem bronchus bifurcates twice, forming three secondary bronchi. These bronchi, along with their surrounding mesenchyme, will form the right upper, middle, and lower lobes. On the left, a single major dichotomous branching occurs, forming the upper lobar bronchus, whereas the main bronchus terminates as the left lower lobe. Another round of dichotomous branching leads to the development of 18 total tertiary branches called bronchopulmonary segments, 10 on the right and 8 on the left. These ultimately form a bronchial tree in the mature fetus, as shown in Figure 2-5.
Dichotomous branching at the terminus of each tertiary bronchus proceeds throughout the remainder of the pseudo- glandular period. Most of this branching takes place between weeks 10 and 16. By the end of the 16th week, Bucher and Reid21 were able to identify between 4 and 25 generations of conducting airways, depending on the location of the segment. This subsegmental branching continues into the beginning of week 17. At this point, all nonrespiratory airway elements have formed, but the terminal bronchi, respiratory bronchi, and acinar units are difficult to delineate. These terminal sac structures will be defined during the canalicular period of development.
In addition to conducting airway growth, the pseudoglandular period also encompasses important developmental milestones for several surrounding structures, including the chest wall, mediastinum, and diaphragm. During weeks 8 through 10, the diaphragm is developing through a complex set of
mesenchymal layer fusions, as noted by Boyden.12 As the lungs grow first dorsally and then inferiorly, the pleural spaces descend. During this descent, Bremer15 observed that two layers of innermost chest wall musculature are “borrowed” from the chest wall. The first, a dorsal layer, forms the crura of the diaphragm. The second, an anterolateral layer, merges with the first, and the two fuse as the lumbocostal trigone. These fusions must take place by day 60 of embryonic development, when the intestines return to the peritoneal cavity from the umbilical coelom. Failure of fusion at this point may lead to eventration or abdominal viscus herniation through the foramen of Bochdalek. Such a defect is seen 1 in 2,500 newborns and is commonly known as pulmonary hypoplasia, with the associated diaphragmatic hernia, depending on the severity of the condition, leading to morbidity and mortality. The recent understanding of this condition is that exposure of the pregnant woman to environmental toxicants (cigarette smoke, pesticides, chemical fumes, etc.) during weeks 3 to 4 of the gestational period can lead to impaired development of the lung, heart, and diaphragm in the developing embryo.28,29 Pulmonary hypoplasia can occur without
congenital diaphragmatic hernia under less severe exposure, which is seen in 1 in 800 newborns. This observation counters the earlier theory that the defect in the diaphragm results in hypoplastic lung development, thus suggesting that embryonic exposure to toxicants can lead to defective organogenesis in developing human or animal embryos, depending on the time and dose of exposure.28,29
mesenchymal layer fusions, as noted by Boyden.12 As the lungs grow first dorsally and then inferiorly, the pleural spaces descend. During this descent, Bremer15 observed that two layers of innermost chest wall musculature are “borrowed” from the chest wall. The first, a dorsal layer, forms the crura of the diaphragm. The second, an anterolateral layer, merges with the first, and the two fuse as the lumbocostal trigone. These fusions must take place by day 60 of embryonic development, when the intestines return to the peritoneal cavity from the umbilical coelom. Failure of fusion at this point may lead to eventration or abdominal viscus herniation through the foramen of Bochdalek. Such a defect is seen 1 in 2,500 newborns and is commonly known as pulmonary hypoplasia, with the associated diaphragmatic hernia, depending on the severity of the condition, leading to morbidity and mortality. The recent understanding of this condition is that exposure of the pregnant woman to environmental toxicants (cigarette smoke, pesticides, chemical fumes, etc.) during weeks 3 to 4 of the gestational period can lead to impaired development of the lung, heart, and diaphragm in the developing embryo.28,29 Pulmonary hypoplasia can occur without
congenital diaphragmatic hernia under less severe exposure, which is seen in 1 in 800 newborns. This observation counters the earlier theory that the defect in the diaphragm results in hypoplastic lung development, thus suggesting that embryonic exposure to toxicants can lead to defective organogenesis in developing human or animal embryos, depending on the time and dose of exposure.28,29
Table 2-2 Comparative Stages of Lung Development | ||||||||||||||||||||||||||||||
---|---|---|---|---|---|---|---|---|---|---|---|---|---|---|---|---|---|---|---|---|---|---|---|---|---|---|---|---|---|---|
|
Canalicular Period: Weeks 17 to 28
Overlap as proximal segments mature faster than distal ones.
Lumens of bronchi and bronchioles become larger.
Tissue becomes more vascular.
Respiratory bronchioles and alveolar ducts develop.
Some terminal sacs develop.
Respiration is possible at the end of this stage.
The canalicular period encompasses weeks 17 through 26 or, as largely accepted, up to 28 weeks based on Thurlbeck.98 Bucher and Reid21 have confirmed that by the beginning of this period, all axial, nonrespiratory portions of the bronchial tree are present. With the conducting portions of the respiratory and pulmonary vascular system in place, the canalicular period focuses on the capillarization and morphogenesis of the pulmonary acini. By the end of this period, the respiratory system will be capable of gas exchange, with the potential to sustain an air-breathing existence.
Boyden13 showed that distal bronchioles at week 17 terminate in several generations of closely arranged buds. Histologically, the airspaces at this point are composed of large rounded areas, and the intervening connective tissue/mesenchyme is abundant (Fig. 2-6). During the canalicular period, the interstitial tissue between each airspace thins. At the same time, elastic tissue forms around the airspaces. The elastic tissue serves two functions. First, it lends elasticity to the lung, providing the source for passive expiratory force in postnatal life. Second, it helps to demarcate each saccular unit. As the saccular units become delineated, the acinus—composed of one terminal bronchiole, two to four respiratory bronchioles, and four to seven saccules per respiratory bronchiole—can be defined (Fig. 2-7A,B).
During this period, capillaries move toward the adjacent primordia of future airspaces as spaces enlarge and the interstitium shrinks. Boyden13 showed that the apposition of capillaries to the future airspaces begins first at the most distal respiratory units and bronchial branch points. Based on histologic studies, it was first thought that the capillaries actually penetrated the lumens of the air spaces. Elegant studies, first by Low65 using the electron microscope and later by several other developmental biologists, proved that the capillaries insert themselves between cuboidal epithelial cells and near the bronchial lumen, but a thin layer of cytoplasm always intervenes.
As the lung develops further, airway branching continues, along with development of the pulmonary vasculature. The airway cells differentiate into ciliated cells, goblet cells, and basal epithelial cells, all of which are tall columnar cells. As further branching proceeds, the distal regions are lined with a single layer of tall columnar cells. These structures mark the future acini, which are small tubular structures surrounded by mesenchyme (Fig. 2-6). As the tubular structures develop, the mesenchyme around them thins and the columnar epithelial cells become short columnar cells and eventually cuboidal epithelial cells (Fig. 2-8). With thinning of mesenchyme, a loose network of capillaries also develops around the acini. During these stages of development the epithelial cell population in the lung doubles and the cuboidal epithelial cells lining the acini have a cytoplasm that is heavily laden with glycogen. Basally, the acini are lined by the basement membrane (BM), with no interstitial collagen or elastin in this part of the lung. The major distinguishing features between the pseudoglandular period and canalicular period are the development of the pulmonary vasculature and thinning of the mesenchyme (Fig. 2-9).
Toward the end of canalicular period and the beginning of saccular period, simultaneous with saccular capillarization, the acinar endothelium as described by Policard and colleagues79 differentiates into type I and type II pneumocytes. Some of the acinar cuboidal epithelial cells become spindle-shaped by voiding most cell organelles and cytoplasmic glycogen as they become specialized squamous cells for gas exchange; thus they form a microthin membrane for gas exchange between red blood corpuscles in the capillaries lining the alveolar air spaces. These cells are termed type I pneumocytes, and they represent 80% to 90% of the alveolar surface area. At birth, the thickness of the blood–gas barrier formed by fusion of the capillary wall and the type I pneumocyte is as thin as 4 μm (Fig. 2-9).
In contrast to type I pneumocytes, type II pneumocytes retain the glycogen of their cuboidal precursor cells. Type II pneumocytes are about 6 μm in size and produce various phospholipids, including phosphatidylcholine—a precursor of the major surfactant phospholipid dipalmitoyl phosphatidylcholine—which are then stored in the lamellar bodies; these are the phospholipid (surfactant) storage bodies. Type II pneumocytes are readily identified by the presence of these surfactant storage bodies (Fig. 2-10C). Farrell40 pointed out that this phospholipid is produced using the cytidine diphosphocholine (CDP-choline) pathway. The production of surface-active material from this pathway is influenced by a number of neural and hormonal factors, including the presence of surrounding mesenchyme, corticosteroids, and thyroxine.
Along with phospholipid, surfactant contains various carbohydrates, cholesterol, and apoproteins known as surfactant proteins (SP)-A, -B, -C, and -D, where SP-A and SP-D are hydrophilic proteins and SP-B and SP-C are hydrophobic proteins. This material provides a protective lining for the alveoli by creating a monolayer at the air–cell interface. Von Neergaard101 observed that the effect of this monolayer is to reduce surface tension in the alveolus, preventing its collapse during expiration and reducing the elastic property of the lung by at least two-thirds. Brumley and colleagues19 noted that sufficient quantities of surfactant are produced by 24 to 26 weeks to provide the alveolar stability required for gas exchange. Premature infants born at this stage of pulmonary development may, therefore, be viable, although exogenous surfactant is frequently required as a therapeutic intervention to lessen the morbidity and mortality of hyaline membrane disease.
Saccular/Terminal Sac Period: Week 28 to Birth
Many more terminal sacs develop.
Epithelium becomes very thin.
Capillaries bulge into the alveoli.
Type I alveolar cells develop.
Capillary network develops rapidly.
By 20 weeks, type II alveolar cells begin producing surfactant, which permits expansion of the terminal sacs.
A fetus must weigh 1,000 g and be between 26 and 28 weeks before enough surfactant is produced. Both surfactant and a sufficient number of capillaries are necessary to sustain life.
The terminal sac period, which lasts from week 28 until birth, focuses on development of the respiratory saccule. By the beginning of the third trimester, three orders of respiratory bronchi can clearly be seen to originate from each terminal bronchiole. Loosely defined respiratory buds that are present at the beginning of the canalicular period have now developed into several orders of distinct capillarized saccules. Throughout the terminal sac period, the saccules continue to become septated. The septations create thin-walled subsaccular structures that will mature into true alveoli.
This transformation represents a continuum of development from saccule to alveolus, which has created a debate about the exact time when saccules become alveoli. Boyden10,11 believed that the development of alveoli occurred postnatally and was dependent on the force of air movement within the lung. In his schema, therefore, the terminal sac period is present until birth. Thurlbeck98 identified maturing alveoli prenatally during the third trimester and so divides the terminal sac period into saccular and alveolar stages (Figs. 2-7A,B and 2-11). In either case, it is clear that most alveolar morphogenesis occurs postnatally. Based on lung morphometry studies by Weibel103 and Langston and colleagues,61 it is estimated that only 20 to 50 million alveoli, or 8% of alveoli present in adulthood, have formed by 40 weeks’ gestation.
During the third trimester of gestation, respiratory movements have been identified, although they are intermittent. There has been considerable debate about the triggers of these motions, their role in pulmonary alveolar development, and their role in amniotic fluid production. The embryonic diaphragm, innervated by the phrenic nerve, does contract, although in utero this action produces paradoxical respiratory movement with chest contraction and abdominal distention. Windle and associates107 believed that intrauterine respiratory movements were a response to abnormally low fetal oxygenation as sensed by peripheral chemoreceptors. Later, studies conducted by Biscoe5 and Woodrum109 and their colleagues on the lamb fetuses showed that these movements were centrally controlled and do not depend on input from the carotid body chemoreceptors. It is now clear that fetal lung movements are mediated at the level of the brainstem.
The role of fetal lung movements in alveolar development is still debated. At one time, it was thought that these movements created a net inspiration of amniotic fluid and that this
influx helped mold and form alveoli. It is now known that the net movement of fluid is from the lung to the amnion. DeBlasio and colleagues34 demonstrated that aspiration of amniotic fluid does not occur in utero before labor. This fact has important clinical significance. Gluck and coworkers45 noted that the presence of increasing levels of pulmonary surfactant expelled from the lungs into the amniotic milieu during the third trimester allows for calculation of a lecithin-to-sphingomyelin ratio in fluid drawn at amniocentesis. This ratio is an accurate measure of fetal lung maturity and correlates with postnatal resistance to hyaline membrane disease in premature infants.
influx helped mold and form alveoli. It is now known that the net movement of fluid is from the lung to the amnion. DeBlasio and colleagues34 demonstrated that aspiration of amniotic fluid does not occur in utero before labor. This fact has important clinical significance. Gluck and coworkers45 noted that the presence of increasing levels of pulmonary surfactant expelled from the lungs into the amniotic milieu during the third trimester allows for calculation of a lecithin-to-sphingomyelin ratio in fluid drawn at amniocentesis. This ratio is an accurate measure of fetal lung maturity and correlates with postnatal resistance to hyaline membrane disease in premature infants.
Alveolar Period: Late Fetal Period to Childhood
Formation of squamous epithelium to prepare for the air-breathing surface.
During this period respiratory bronchioles end as terminal sacs.
Terminal sacs become alveolar ducts.
Alveoli form after birth.
From the third to the eighth year, alveoli continue to develop.
Lungs at Birth
At birth the lungs are filled with fluid.
Fluid is replaced by air.
Fluid is cleared through mouth and nose, pulmonary capillaries, pulmonary arteries, veins, and lymphatics.
After birth, most growth is in the number of respiratory bronchioles and alveoli and not in an increase in the size of alveoli.
Removal of Liquid from the Fetal Lung
Rapid removal of liquid from potential airspaces is a key step in establishing the timely switch from placental to pulmonary gas exchange at birth.6 A sudden gush of fluid from the mouth often punctuates a baby’s birth, signaling the start of extrauterine life. Although enormous progress has been made in recent years to help clarify how liquid is produced in the fetal lung and how it is removed during and after birth, relatively little attention has been paid as to how this information relates to the human lung during development and how it might be applied therapeutically to alter lung growth before birth or to prevent respiratory distress after birth. Knowledge of how the secretion of epithelial chloride might be enhanced pharmacologically could prove useful in promoting the expansion and growth of the fetal lung in situations known to be associated with pulmonary hypoplasia (congenital diaphragmatic hernia, prolonged oligohydramnios).6
Understanding how alveoli and the underlying capillary network develop and how these mechanisms are disrupted in preterm infants with bronchopulmonary dysplasia (BPD) is critical to developing efficient and effective therapies for lung diseases characterized by alveolar damage. Since the initial description of BPD almost half a century ago, advances in perinatal care have allowed the survival of less mature infants. The disease has not disappeared, but it now affects infants with undeveloped distal airspaces, resulting in an arrest of alveolar development. The histologic changes that occur during normal lung development are well described, but little is known about the signaling mechanisms that regulate saccular and alveolar development (Fig. 2-12). A brief review by Thébaud97 focuses on the recently recognized role of angiogenic growth factors during normal alveolar development, injury, and repair, with particular emphasis on the vascular endothelial growth factor.
As discussed, different phases of lung development are marked by several changes in lung structure, including the changes in epithelial cell shapes. Epithelial cells alter from tall columnar cells in pseudoglandular period to short columnar cells in canalicular period to cuboidal cells in terminal sac period
(Figs. 2-8 and 2-13). Transition from the canalicular to the saccular phase is marked by the development of air sacs, or the terminal sacs. The proliferation rate of the epithelial cells drops at this stage and differentiation is predominant. The intracellular glycogen in the cuboidal epithelial cells is reduced dramatically and the formation of lamellar bodies begins, followed by their secretion. From this stage on, the markers of alveolar epithelial cells—such as surfactant proteins, several membrane-associated proteins, and cytokeratins—can be distinctly detected. Gradually, the thinning of the mesenchyme and differentiation of the epithelial cells into the cuboidal epithelial cells, followed by further differentiation into type I cells in addition to the type II cells, occurs in air-breathing lungs at birth, an alveolar stage of the lung. At this stage there are no true alveoli; however, the type II cells secrete lamellar bodies, i.e., surfactant, that prepares the lung for becoming an air-breathing lung.
(Figs. 2-8 and 2-13). Transition from the canalicular to the saccular phase is marked by the development of air sacs, or the terminal sacs. The proliferation rate of the epithelial cells drops at this stage and differentiation is predominant. The intracellular glycogen in the cuboidal epithelial cells is reduced dramatically and the formation of lamellar bodies begins, followed by their secretion. From this stage on, the markers of alveolar epithelial cells—such as surfactant proteins, several membrane-associated proteins, and cytokeratins—can be distinctly detected. Gradually, the thinning of the mesenchyme and differentiation of the epithelial cells into the cuboidal epithelial cells, followed by further differentiation into type I cells in addition to the type II cells, occurs in air-breathing lungs at birth, an alveolar stage of the lung. At this stage there are no true alveoli; however, the type II cells secrete lamellar bodies, i.e., surfactant, that prepares the lung for becoming an air-breathing lung.
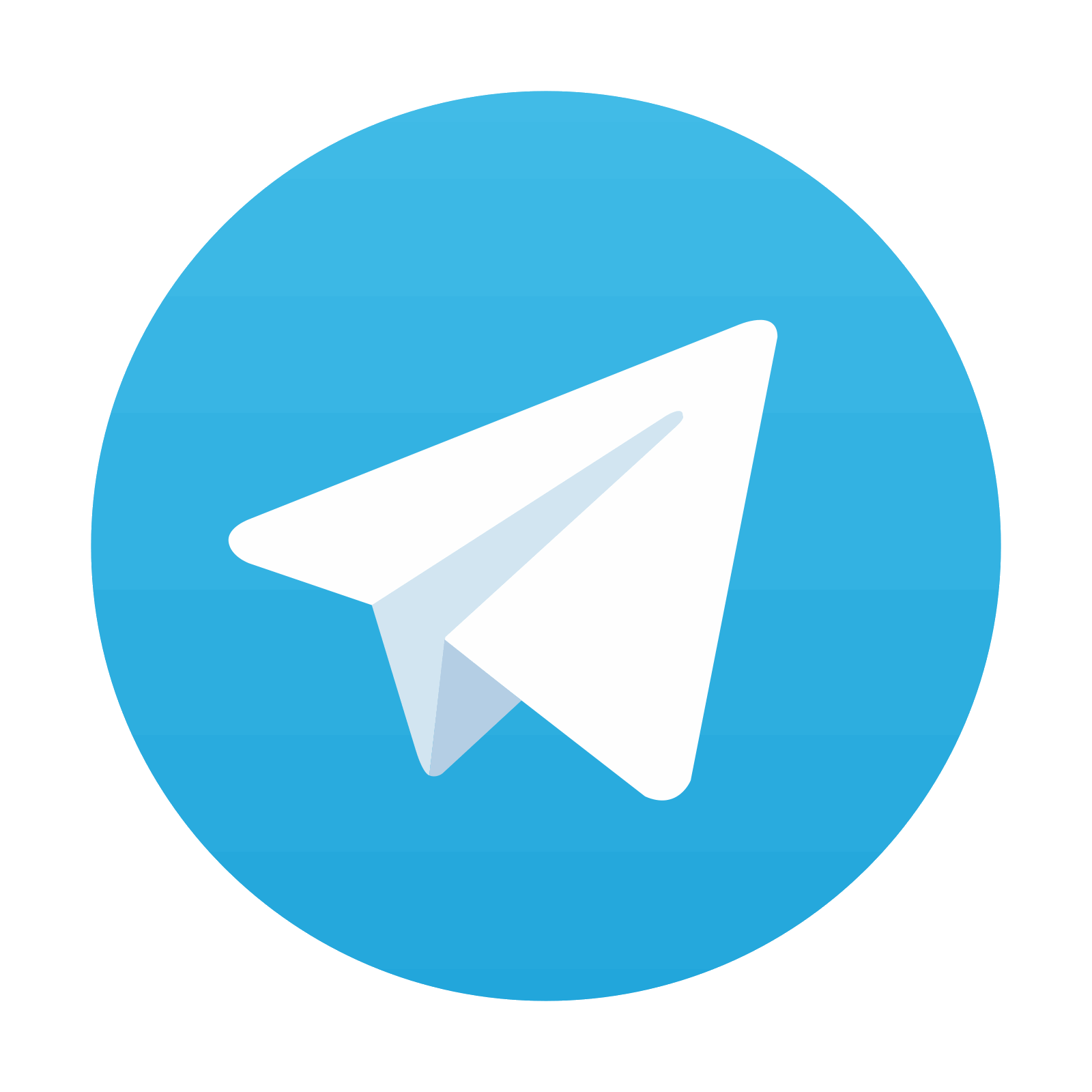
Stay updated, free articles. Join our Telegram channel
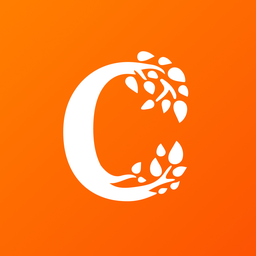
Full access? Get Clinical Tree
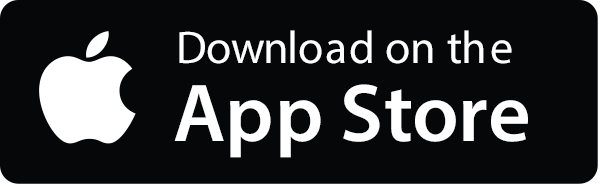
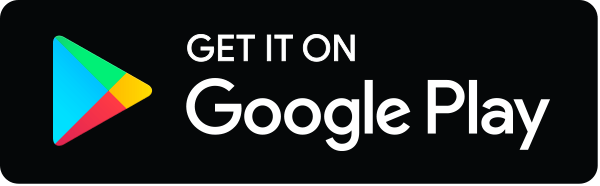