Background
Assessment of left ventricular rotational mechanics and myocardial deformation may provide new insight into both systolic and diastolic function. However, the effects of increasing afterload on these measures of cardiac function are poorly understood. The aim of this study was to identify the changes in left ventricular function and rotational mechanics during increasing pharmacologic afterload.
Methods
In 14 anesthetized rabbits, two-dimensional speckle-tracking echocardiographic images and left ventricular pressure-volume loops were acquired at baseline and during norepinephrine, phenylephrine, and vasopressin infusion at increasing doses. Maximal ventricular elastance, arterial elastance, ventricular-arterial coupling, dP/dt, the time constant of relaxation, and other hemodynamic parameters were determined.
Results
An increase in dP/dt max with norepinephrine and phenylephrine and a decrease with vasopressin at escalating doses were detected. Ventricular-arterial coupling was preserved with norepinephrine and phenylephrine but decreased with vasopressin ( P < .05). Apical rotation, rotational rate, and strain were preserved during the norepinephrine and phenylephrine infusions but were reduced with vasopressin ( P < .05). Apical rotation and circumferential strain were significantly correlated with both ventricular-arterial coupling ( r = 0.84 and r = 0.81) and dP/dt max ( r = −0.81 and r = −0.77). High-dose vasopressin decreased the diastolic time constant of relaxation and dP/dt min while reducing apical untwisting rate.
Conclusions
Pharmacologic increases in afterload with vasopressin resulted in greater derangements in ventricular-arterial coupling and cardiac performance compared with norepinephrine and phenylephrine. Rotation and strain correlated well with invasively determined measures and can be used to assess afterload-induced alteration in cardiac function.
In recent years, the introduction of speckle-tracking echocardiography (STE) has renewed interest in using indices of myocardial deformation to assess ventricular function. Left ventricular (LV) myocardial strain and LV rotational mechanics are now readily characterized and provide new insight into both systolic and diastolic function. In the critical care and perioperative clinical settings, pharmacologically induced elevation of afterload is often used to improve systemic perfusion. Although elevation of arterial blood pressure is evident with these drugs, the effects on cardiac performance can vary and are difficult to define. In part, the variability is due to differential actions on cardiovascular receptors that create imbalance in relative states of ventricular afterload and contractility, so-called ventricular-arterial coupling. The effects of increasing afterload and altered ventricular-arterial coupling on STE-derived strain and rotational parameters have not yet been described and may reflect changes in systolic and diastolic ventricular performance.
In this study, our aim was to identify changes in LV strain and rotational indices that reflect effects of increasing afterload on systolic and diastolic function and to compare them to intraventricular pressure-volume relationships. We hypothesized that in vivo afterload increase by three clinically used pharmacologic agents, norepinephrine, phenylephrine, and vasopressin, alters LV rotational and strain indices in accordance with their effects on myocardial function and ventricular-arterial coupling.
Methods
All animals received humane treatment in compliance with the 1996 National Research Council Guide for the Care and Use of Laboratory Animals after approval by the University of California, Los Angeles, Division of Laboratory Animal Medicine and the Animal Research Committee.
Experimental Procedures
Fourteen rabbits (mean weight, 3.07 ± 0.31 kg) were anesthetized with intramuscular ketamine (15 mg/kg) and intramuscular xylazine (1 mg/kg). Anesthesia was maintained with isoflurane (1%–2%) with bolus injections of fentanyl (5 μg/kg) and vecuronium (0.1 mg/kg). Respiratory support was initiated after endotracheal intubation with pressure-controlled ventilation (Servo 900C; Siemens-Elema, Solna, Sweden) (inspired oxygen fraction, 1.0). Tidal volume (10–15 mL/kg) and breathing rate (22–28 breaths/min) were adjusted to maintain end-tidal carbon dioxide at 30 to 34 mm Hg. Body temperature was maintained at 37°C using a heating blanket; isotonic saline solution was infused through a central venous line at a rate of 3–5 mL/kg per hour. Arterial oxygen saturation and an electrocardiogram were continuously monitored. The femoral artery was cannulated to monitor mean arterial pressure (MAP). One femoral vein and one jugular vein were cannulated for intravenous infusion of fluids and drugs.
After midline sternotomy and longitudinal pericardial incision, animals were heparinized (100 U/kg), and the left ventricle was instrumented with a 3-Fr dual-field micromanometer-tipped pressure-conductance catheter (Millar Instruments, Inc., Houston, TX) through the LV apex. Correct position of the catheter was confirmed echocardiographically. A snare was placed around the inferior vena cava for preload intervention.
Data Acquisition
Invasive Hemodynamic Monitoring with Pressure-Volume Loop Analysis
Hemodynamic pressure-volume data were digitally recorded during caval occlusion with suspension of mechanical respiration (10–15 cardiac cycles). Heart rate, end-systolic and end-diastolic pressures, end-systolic and end-diastolic volumes, stroke volume, ejection fraction, and cardiac output (CO) are reported. As a measure of afterload, arterial elastance (E A ) was calculated as end-systolic pressure divided by stroke volume. LV pressures and its derivatives (dP/dt max and dP/dt min ) and the time constant of relaxation (τ) were analyzed using PVAN Ultra Analysis Software version 1.0 (Millar Instruments, Inc.). LV end-systolic elastance (E max ) was assessed as a load-independent measure of contractility and was determined by the slope of the linear end-systolic pressure–volume relation using PVAN Ultra Analysis Software. The ratio of E max to E A was determined to express the balance between ventricular contractility and arterial resistance (ventricular-arterial coupling).
Echocardiographic Imaging
Open-chest epicardial echocardiography was performed using a GE Vivid 7 Dimension system (GE Vingmed Ultrasound AS, Horten, Norway) with a 10-MHz transducer and tissue velocity and Doppler imaging capabilities. Short-axis views at the apical, midpapillary, and basal levels were obtained with digitized two-dimensional and color-coded tissue velocity imaging (two-dimensional frame rate, 120–150 frames/sec). Apical short-axis views were obtained by moving the probe apically from a midpapillary view until the papillary muscles were no longer visible. Basal short-axis views were obtained at the level of the mitral valve. Apical four-chamber views including the mitral lateral annulus were recorded using color-coded tissue velocity imaging (tissue velocity imaging frame rate > 150 frames/sec; pulse repetition frequency, 4.5 kHz; velocity range, ± 16 cm/sec). Because of the apical cannulation site, the apical four-chamber views were modified to maintain alignment with the mitral annulus for analysis of tissue velocity. This was accomplished with transducer placement slightly anterior to the apex. Two-dimensional short-axis views of the base and apex were analyzed using speckle-tracking software (EchoPAC PC version 8.0; GE Vingmed Ultrasound AS) for rotation, rotational rate, and peak untwisting rate; the midpapillary views were analyzed for global circumferential strain (ϵ Circ ) and strain rate (SR Circ ) as well as average radial strain and strain rate. By convention, counterclockwise rotation as viewed from the LV apex was reported as a positive value. Fractional area change was determined, and tissue velocity images were analyzed for peak mitral annular velocities during systole (s′) and early diastole (e′).
Experimental Protocols
The animals were stabilized for a 30-min period before baseline measurements. After baseline hemodynamic and echocardiographic data were recorded, infusions of norepinephrine, phenylephrine, and vasopressin were initiated.
The order of drug infusions was randomized, and doses were adjusted to achieve a predefined increase in MAP: low (MAP 10%–15% above baseline), medium (MAP 20%–25% above baseline), and high (MAP >30% over baseline) levels. Hemodynamic parameters were allowed to stabilize for approximately 15 min at each dose before data acquisition; after the cessation of infusion of each drug, hemodynamic values were allowed to return to baseline before starting the next infusion. All animals were euthanized at the end of the study with pentobarbital (100 mg/kg) and potassium chloride solution injection intravenously.
Statistical Analysis
Analyses were performed using SigmaStat version 3.1 (Systat Software, San Jose, CA). Results are expressed as mean ± SD. The hemodynamic and echocardiographic measurements were compared in the experimental stages using repeated-measures analysis of variance with pairwise multiple comparison procedures performed using the Holm-Sidak method. The relationship between hemodynamic and echocardiographic variables was analyzed using linear regression analysis. P values < .05 were considered statistically significant. Importantly, hemodynamic analysis was performed by investigators blinded to the results of the echocardiographic data and to the order of pharmacologic drug infusions.
Interobserver and Intraobserver Reproducibility
Two independent observers processed echocardiography images for seven randomly selected study animals for apical rotation and circumferential strain. Each observer analyzed the same image twice. Intraobserver variability was determined by having one observer repeat the measurements 1 month after the initial analysis. Interobserver and intraobserver variability was assessed using intraclass correlation coefficients with 95% confidence intervals, as we demonstrated previously.
Results
Low, middle, and high dose ranges for the vasoconstrictors were 0.05 to 0.15, 0.2 to 0.4, and 0.5 to 1.0 μg/kg/min for norepinephrine; 0.5 to 1.5, 2 to 4, and 5 to 10 μg/kg/min for phenylephrine; and 0.5 to 1.5, 2 to 3, and 4 to 5 mU/kg/min for vasopressin, respectively. Hemodynamic and echocardiographic data at baseline and during drug infusions are reported in Tables 1 and 2 . Escalating doses of all vasoconstrictors increased end-systolic pressure and E A and decreased heart rate. The effect on heart rate was most pronounced with phenylephrine and vasopressin, reaching statistical significance with the high doses in those groups. The changes in E A compared with baseline were significant ( P < .05) in the high-dose phenylephrine and vasopressin groups, with the greatest increase in the vasopressin group.
Variable | Control | Norepinephrine | Phenylephrine | Vasopressin | ||||||
---|---|---|---|---|---|---|---|---|---|---|
Low | Medium | High | Low | Medium | High | Low | Medium | High | ||
HR (beats/min) | 179 ± 25 | 165 ± 21 | 156 ± 22 | 150 ± 34 | 174 ± 25 | 158 ± 30 | 133 ± 34 ∗ | 154 ± 29 | 144 ± 31 ∗ | 138 ± 36 ∗ |
ESP (mm Hg) | 66 ± 9 | 73 ± 9 | 82 ± 11 ∗ | 98 ± 9 ∗ | 75 ± 8 | 84 ± 7 ∗ | 106 ± 13 ∗ | 75 ± 12 | 82 ± 9 ∗ | 100 ± 20 ∗ |
EDP (mm Hg) | 6.2 ± 4.8 | 7 ± 3.1 | 6.4 ± 3.4 | 8.2 ± 4.5 | 8.2 ± 5 | 6.9 ± 3.9 | 7.9 ± 3.6 | 8.1 ± 3.6 | 8.2 ± 3.1 | 9.8 ± 3.9 |
ESV (mL) | 1.8 ± 0.5 | 1.7 ± 0.8 | 2.0 ± 0.6 | 1.8 ± 0.6 | 1.7 ± 0.8 | 1.9 ± 0.5 | 1.8 ± 0.6 | 2.1 ± 0.9 | 2.1 ± 0.8 | 2.3 ± 0.8 |
EDV (mL) | 3.7 ± 0.5 | 4.0 ± 0.5 | 4.2 ± 0.3 | 4.1 ± 0.4 | 3.8 ± 0.4 | 4.0 ± 0.4 | 3.9 ± 0.4 | 4.0 ± 0.6 | 3.9 ± 0.5 | 3.8 ± 0.3 |
SV (mL) | 1.9 ± 0.7 | 2.1 ± 0.8 | 2.2 ± 0.8 | 2.3 ± 0.7 | 1.9 ± 0.5 | 2.0 ± 0.7 | 2.1 ± 0.6 | 1.9 ± 0.7 | 1.8 ± 0.7 | 1.5 ± 0.7 |
E A (mm Hg/mL) | 39 ± 14 | 40 ± 14 | 42 ± 15 | 47 ± 15 | 41 ± 14 | 46 ± 19 | 54 ± 20 ∗ | 42 ± 14 | 53 ± 23 | 77 ± 27 ∗,†,‡ |
CO (mL) | 326 ± 124 | 306 ± 176 | 341 ± 125 | 352 ± 115 | 322 ± 99 | 299 ± 77 | 260 ± 72 | 304 ± 127 | 262 ± 137 ∗,‡ | 211 ± 128 ∗,‡ |
EF (%) | 49 ± 15 | 51 ± 16 | 52 ± 15 | 56 ± 15 | 49 ± 14 | 50 ± 15 | 51 ± 15 | 50 ± 18 | 47 ± 19 | 39 ± 20 |
Variable | Control | Norepinephrine | Phenylephrine | Vasopressin | ||||||
---|---|---|---|---|---|---|---|---|---|---|
Low | Medium | High | Low | Medium | High | Low | Medium | High | ||
Apical | ||||||||||
Rotation (°) | 14.0 ± 2.7 | 12.7 ± 2.5 | 12.7 ± 3.7 | 13.5 ± 3.9 | 14.0 ± 4.2 | 13.6 ± 3.9 | 13.7 ± 5.1 | 11.0 ± 2.6 | 9.4 ± 5.2 ∗ | 6.9 ± 3.5 ∗,‡,§ |
RR (°/sec) | 93 ± 22 | 91 ± 19 | 90 ± 29 | 97 ± 36 | 95 ± 35 | 83 ± 26 | 76 ± 32 | 72.2 ± 21 | 70.0 ± 38 | 48 ± 18 ∗,§ |
Basal | ||||||||||
Rotation (°) | −5.8 ± 1.0 | −5.1 ± 2.2 | −4.23 ± 2.1 | −5.6 ± 3.2 | −5.9 ± 1.6 | −4.6 ± 1.6 | −4.8 ± 2.0 | −5.4 ± 1.1 | −4.2 ± 2.3 | −4.7 ± 2.5 |
RR (°/sec) | −105 ± 27 | −88 ± 25 | −85 ± 15 | −88 ± 40 | −105 ± 20 | −92 ± 26 | −75 ± 29 ∗ | −105 ± 17 | −87 ± 47 | −70 ± 22 ∗ |
Midpapillary | ||||||||||
ϵ Circ (%) | −20.2 ± 2.8 | −19.9 ± 6.1 | −19.8 ± 4.1 | −19.8 ± 3.4 | −20.0 ± 3.0 | −20.7 ± 3.9 | −20.0 ± 3.9 | −19.4 ± 4.7 | −16.0 ± 5.6 ∗ | −13.4 ± 3.1 ∗,‡,§ |
SR Circ (%/sec) | −2.4 ± 0.6 | −2.4 ± 0.7 | −2.3 ± 0.5 | −2.3 ± 0.5 | −2.4 ± 0.6 | −2.3 ± 0.6 | −1.9 ± 0.4 ∗ | −2.2 ± 0.4 | −2.0 ± 0.5 | −1.5 ± 0.3 ∗,‡,§ |
ϵ Rad (%) | 48.1 ± 12.3 | 50.4 ± 10.9 | 54.9 ± 14.6 | 52.0 ± 13.0 | 48.8 ± 12.3 | 51.7 ± 9.5 | 48.1 ± 12.0 | 44.9 ± 13.5 | 38.6 ± 10.9 ‡,§ | 29.0 ± 15.7 ∗,‡,§ |
SR Rad (%/sec) | 3.6 ± 0.8 | 3.2 ± 0.69 | 3.4 ± 0.8 | 3.2 ± 0.5 | 3.4 ± 0.7 | 3.1 ± 0.6 | 3.0 ± 0.8 | 3.2 ± 0.5 | 3.0 ± 0.6 | 2.6 ± 0.5 ∗,‡,§ |
Net LV twist (°) | 20.2 ± 2.9 | 18.7 ± 3.9 | 16.8 ± 5.0 | 19.3 ± 6.2 | 20.8 ± 5.1 | 19.1 ± 4.9 | 18.9 ± 6.0 | 17.8 ± 3.1 | 15.3 ± 6.7 ∗ | 12.6 ± 4.2 ∗,‡,§ |
FAC (%) | 60 ± 6 | 62 ± 8 | 61 ± 8 | 59 ± 11 | 62 ± 5 | 57 ± 12 | 59 ± 7 | 58 ± 6 | 53 ± 11 ∗,§ | 46 ± 7 ∗,‡,§ |
s′ (cm/sec) | 4.5 ± 0.8 | 4.32 ± 1.1 | 5 ± 1.8 | 4.6 ± 1.3 | 4.7 ± 06 | 4.8 ± 1.1 | 4.1 ± 0.6 | 4.67 ± 0.9 | 3.2 ± 0.9 ∗,‡,§ | 2.8 ± 0.5 ∗,†,‡,§ |
‡ P < .05 versus phenylephrine.
Systolic LV performance was preserved with low, medium, and high doses of phenylephrine and norepinephrine, as demonstrated by either no change or a slight increase in E max and increases in dP/dt max with maintained stroke volume and CO ( Tables 1 and 3 ). Although E max was unchanged for vasopressin, diminished LV performance was demonstrated during high-dose vasopressin by a fall in CO and reduced dP/dt max compared with baseline and high doses of norepinephrine and phenylephrine. Consistent with these findings, the peak lateral mitral annular velocity (s′) was preserved with norepinephrine and phenylephrine but reduced with vasopressin (baseline vs high, 4.5 vs 2.8 cm/sec; P < .05; Table 2 ). The ventricular-arterial coupling ratio, E max /E A , was determined using the elastance values of the respective chambers ( Table 3 , Figure 1 ). This parameter demonstrated modest increases with norepinephrine infusion ( P = NS), modest decreases with phenylephrine infusion ( P = NS), and decreases with vasopressin administration, which were significant at high doses ( P < .05).
Variable | Control | Norepinephrine | Phenylephrine | Vasopressin | ||||||
---|---|---|---|---|---|---|---|---|---|---|
Low | Medium | High | Low | Medium | High | Low | Medium | High | ||
dP/dt max (mm Hg/sec) | 3,351 ± 1,038 | 4,005 ± 1,304 | 4,262 ± 1,308 ∗ | 4,981 ± 1,951 ∗,† | 3,809 ± 1,216 | 3,953 ± 1,399 ∗ | 4,752 ± 1,404 ∗,† | 3,138 ± 850 | 2,957 ± 1,245 ‡,§ | 2,707 ± 1,031 ∗,‡,§ |
E max (mm Hg/mL) | 32.4 ± 14.8 | 40.7 ± 21 | 38 ± 12.4 | 43.3 ± 14.5 | 28.9 ± 10.6 | 32.2 ± 12.4 | 33 ± 16 | 34.9 ± 15.9 | 30.1 ± 17.8 | 22.3 ± 10.1 ∗,†,‡,§ |
E max /E A | 0.8 ± 0.3 | 1.0 ± 0.3 | 0.9 ± 0.2 | 0.9 ± 0.2 | 0.7 ± 0.2 | 0.7 ± 0.2 | 0.6 ± 0.2 § | 0.8 ± 0.2 | 0.6 ± 0.2 | 0.3 ± 0.1 ∗,†,‡,§ |
‡ P < .05 versus phenylephrine.
At the apex of the heart, magnitude of rotation and rotational rates were preserved during the norepinephrine and phenylephrine infusions. With vasopressin, a significant decrement in rotational rates was observed in the high-dose group, and apical rotation was reduced at medium and high doses (14.0° vs 9.4° and 6.9°, P < .05; Figure 2 , Table 2 ). At the level of the base of the heart, the magnitude of rotation was unchanged; systolic rotational rate was decreased with high-dose phenylephrine and vasopressin. The reduction in net LV twist, defined as apical rotation minus basal rotation, was statistically significant at the medium and high doses of vasopressin ( P < .05). A decrement in fractional area change was noted only with high-dose vasopressin.
STE-derived ϵ Circ and SR Circ were unchanged with norepinephrine and phenylephrine, with the exception of a small reduction in SR Circ in the high-dose phenylephrine group. With vasopressin, reductions occurred with escalating doses (baseline vs high-dose vasopressin: ϵ Circ , −20.2% vs −13.4%; SR Circ , −2.43 vs −1.5 %/sec; P < .05). Changes in radial strain parameters were consistent, showing reduction with high-dose vasopressin (baseline vs high-dose vasopressin: average radial strain, 48.1% vs 29.0%; average radial strain rate, 3.6 vs 2.6 %/sec; P < .05).
Strong correlations between apical rotation and E max /E A and dP/dt max ( r = 0.84 and r = 0.81, respectively, P < .05) are reported in Table 4 . Global circumferential strain also correlated well with E max /E A and dP/dt max ( r = −0.81 and r = −0.77, respectively, P < .05); E max /E A was correlated with the apical rotational rates as well as with the circumferential strain rate ( r = 0.69 and r = −0.68, respectively, P < .05). In addition, average radial strain and radial strain rate demonstrated strong correlations with E max /E A ( r = 0.87 and r = 0.73, respectively, P < .05) and a weaker correlation with dP/dt max .
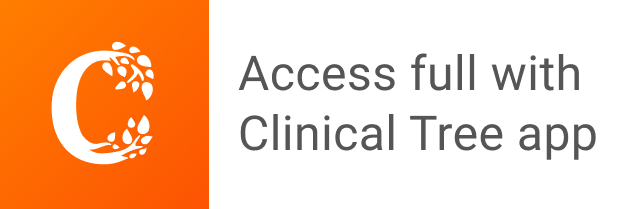