Background
The aim of this study was to examine the occurrence of intra–left ventricular (LV) dyssynchrony in obese versus nonobese subjects without known cardiac disease using Velocity Vector Imaging (VVI).
Methods
One hundred ninety consecutive subjects with no known cardiac disease had their echocardiograms analyzed using VVI after excluding subjects with QRS durations > 120 msec or LV ejection fractions < 55%. Study subjects were divided into two groups on the basis of body mass index: obese (>30 kg/m 2 ) and nonobese (<30 kg/m 2 ).
Results
The final cohort included 136 subjects (74 obese; 32% women; mean age, 55 ± 16 years). The occurrence of intra–LV dyssynchrony was higher in the obese group compared with the nonobese group.
Conclusions
There was an increased prevalence of intra–LV dyssynchrony in obese subjects, especially longitudinal and radial dyssynchrony. This dyssynchrony may signal a mechanism by which obesity predisposes to the development of heart failure.
Obesity is a modern epidemic, with >60 million adults affected in the United States alone. Obesity is an important risk factor for heart failure in both men and women. Increased body mass index (BMI) has been reported in 11% and 14% of men and women with heart failure, respectively. Left ventricular (LV) hypertrophy and dilatation, which are known precursors of heart failure, are associated with obesity. Also, obesity is associated with altered LV remodeling, possibly due to increased hemodynamic load, neurohormonal activation, and increased cytokine production. Myocardial triglyceride content appears to increase progressively with BMI. Recent experimental investigations suggest cardiac steatosis (excessive accumulation of cytosolic triglycerides in the myocardial cells), increased myocardial fibrosis, lipoapoptosis, and the activation of certain cardiac genes may underlie obesity cardiomyopathy. Recent studies using positron emission tomography have found that in obese young women, insulin resistance and obesity are related to alterations in fatty acid metabolism, which could play a role in decreased cardiac performance. Whatever these intricate and complex molecular mechanisms may be, evidence suggests that longstanding obesity results in LV structural and functional alterations, producing volume overload, eccentric LV hypertrophy, systolic and diastolic dysfunction, and heart failure.
Marfella et al. demonstrated a higher occurrence of interventricular dyssynchrony in obese subjects using two-dimensional (2D) echocardiography with Doppler. To our knowledge, there have been no studies thus far examining the incidence of intra–LV dyssynchrony in obese subjects who do not have histories of significant cardiac disease.
Myocardial contraction and relaxation are complex processes involving longitudinal, circumferential, radial, and torsional forces. Velocity Vector Imaging (VVI; Siemens Medical Solutions USA, Inc., Mountain View, CA) is a novel technique that uses myocardial speckle tracking to assess myocardial mechanics from 2D echocardiography. VVI uses an algorithm that automatically tracks motion of the tissue-cavity border and motion of reference points (mitral annulus), displaying tissue motion, direction, and velocity ( Figure 1 ). Unlike Doppler tissue imaging, VVI measures velocities independent of transducer angle. Also, in a recent study, Lim et al. demonstrated that the accuracy of Doppler tissue imaging in assessing LV wall regional motion is limited in dilated ventricles and probably affects LV dyssynchrony measurement.

Our aim was to examine the prevalence of intra–LV dyssynchrony in obese subjects with no histories of cardiac disease and compare them with nonobese controls using VVI.
Methods
Five hundred consecutive subjects who underwent 2D echocardiography at Albert Einstein Medical Center in Philadelphia between November 2008 and March 2009 on an Acuson Sequoia C512 (Siemens Medical Solutions, Inc.) were screened. Three hundred ten subjects were excluded with the following exclusion criteria: (1) history of coronary artery disease; (2) LV ejection fraction (LVEF) < 55%; (3) diastolic dysfunction greater than grade 1 (mitral early to late diastolic inflow peak velocity ratio > 0.8, deceleration time of the mitral inflow < 200 msec, isovolumetric relaxation time < 60 msec, pulmonary venous systolic to diastolic peak velocity ratio < 1, and mitral early inflow to early diastolic annular septal tissue peak velocity ratio > 9, as listed in American Society of Echocardiography criteria ); (4) QRS duration > 120 msec; (5) moderate or severe valvular heart disease (using Doppler echocardiographic parameters: central jet > 4 cm 2 or jet area > 20% of left atrial area for mitral regurgitation, central jet width > 25% or vena contracta > 0.3 cm 2 or pressure half-time < 500 msec for aortic regurgitation, central jet area > 5 cm or proximal isovelocity surface radius > 0.5 cm for tricuspid regurgitation, jet size by color Doppler > 10 mm for pulmonary regurgitation for regurgitant lesions ; mean gradient > 20 mm Hg or aortic valve area < 1.5 cm 2 or aortic jet velocity > 3 m/sec for aortic stenosis, mitral valve area < 1.5 cm 2 or mean gradient > 5 mm Hg for mitral stenosis, tricuspid valve area < 1 cm 2 or mean gradient > 5 mm Hg or inflow time-velocity time integral > 60 cm or pressure half-time > 190 msec for tricuspid stenosis, peak velocity > 3 m/sec or peak gradient > 36 mm Hg for pulmonic stenosis for stenotic lesions ); (6) pacemaker; (7) hypertrophic cardiomyopathy; (8) pericardial effusion or disease; (9) poor-quality images in which the myocardium was not visible; and (10) admission to the intensive care unit. Subjects were divided into two groups on the basis of BMI: (1) obese (BMI ≥ 30 kg/m 2 ) and (2) nonobese (BMI < 30 kg/m 2 ). We also compared morbidly obese subjects (BMI ≥ 40 kg/m 2 ) with obese subjects (BMI ≥ 30 and < 40 kg/m 2 ).
VVI was performed using the Acuson Sequoia C512. Images were captured using frame rates used for traditional 2D echocardiography (30–60 frames/sec). VVI uses a series of tracking algorithms whose details are described elsewhere. In brief, the endocardial-myocardial interface is traced manually in a single frame on a digital cine loop. When the image is processed, a complex algorithm tracks each pixel, and the myocardial velocity vectors are displayed in cine format. The lengths of the vectors are proportional to the magnitude of velocity, and the direction of the arrows corresponds to the direction of myocardial motion. One cardiac cycle was analyzed if the RR intervals were regular, and an average of three beats was used if RR intervals were irregular. Apical four-chamber, two-chamber, and short-axis views at the papillary muscle level were studied offline. In the apical four-chamber and two-chamber views, a trace was made (along the endocardial-myocardial interface) from the septal to lateral mitral annulus and from the inferior to anterior mitral annulus, respectively. In the short-axis view at the level of papillary muscles, a circumferential trace was made starting at the 12 o’clock position and ending at the same point in a clockwise direction, excluding the papillary muscles. Approximately one point per myocardial segment was used to draw the trace. A point of reference was placed at the apex in the two-chamber and four-chamber views to calculate longitudinal velocities and strain. The point of reference was moved to the LV cavity to calculate radial velocities. In the short-axis view, the point of reference was at the center of the left ventricle to calculate circumferential velocities and strain. Longitudinal velocity, longitudinal strain, and radial velocities were measured at the basal septal, basal lateral, basal anterior, and basal inferior walls in the apical four-chamber and two-chamber views. The circumferential velocities and strain were measured in the short-axis view at the papillary muscle level. Time to peak velocities and strain were calculated from the onset of the QRS complex to the peak systolic velocity or peak strain, respectively, during the ejection phase. We defined mechanical dyssynchrony as longitudinal opposing wall delay > 75 msec by VVI on the basis of a prior study. Because there are no published criteria for circumferential dyssynchrony and because we were looking at the maximum delay between all six segments in the short-axis view, not just the opposing wall delays, we used a higher number (maximum delay ≥ 100 msec) to define circumferential dyssynchrony. We used a value of 75 msec for septal–to–lateral wall radial delay. Examples of longitudinal and circumferential LV dyssynchrony analysis using the above-mentioned VVI technique are illustrated in Figures 2 and 3 , respectively.


Patient demographics, clinical characteristics, hemodynamic measurements, laboratory data, echocardiographic parameters, and electrocardiographic data were collected ( Tables 1 and 2 ). Patient demographics collected were age, gender, and race. Clinical characteristics collected were any history of hypertension (blood pressure > 140/90 mm Hg as defined by the seventh report of the Joint National Committee on Prevention, Detection, Evaluation, and Treatment of High Blood Pressure or use of antihypertensive agents); diabetes mellitus (fasting plasma glucose > 126 mg/dL per the American Diabetes Association or receipt of antidiabetic treatment); history of transient ischemic attack, ischemic stroke, or intracranial hemorrhage; hypercholesterolemia (low-density lipoprotein level > 130 mg/d per the National Cholesterol Education Program or statin use); a diagnosis of obstructive sleep apnea ; a diagnosis of stable chronic obstructive pulmonary disease ; and hemodialysis status. Hemodynamic measurements recorded were heart rate and systolic and diastolic blood pressure, recorded immediately before performing echocardiography. Laboratory data obtained were hemoglobin and creatinine, which were assessed closest to the time of echocardiography. QRS duration was recorded from the electrocardiogram. All echocardiographic measurements were based on American Society of Echocardiography guidelines. LV diastolic dimension, septal wall thickness, posterior wall thickness, left atrial diameter, and LV mass were measured using 2D-guided M-mode echocardiography, assuming that the left ventricle is a prolate ellipse in the parasternal long-axis acoustic window. LV mass was indexed to body surface area. Diastolic dysfunction and the grade of dysfunction, LVEF (calculated using the modified Simpson’s rule ), and pulmonary artery systolic pressures were measured.
Variable | Obese ( n = 74) | Nonobese ( n = 62) | P |
---|---|---|---|
Age (y) | 53 ± 13 | 57 ± 18 | .13 |
Men | 22 (29%) | 22 (35%) | .58 |
African Americans | 64 (86%) | 40 (65%) | <.01 |
Hypertension | 57 (77%) | 39 (63%) | .09 |
Diabetes mellitus | 26 (35%) | 17 (27%) | .36 |
Hypercholesterolemia | 20 (27%) | 14 (23%) | .69 |
History of TIA or stroke | 3 (4%) | 3 (5%) | .54 |
Smoker | 18 (24%) | 19 (31%) | .44 |
COPD | 2 (3%) | 4 (6%) | .41 |
Known OSA ∗ | 1 (1%) | 3 (5%) | .62 |
On hemodialysis | 2 (3%) | 4 (6%) | .41 |
Hemoglobin (g/dL) | 11.9 ± 2.5 | 12 ± 1.7 | .56 |
Creatinine (mg/dL) | 1.4 ± 1.4 | 1.8 ± 2.7 | .26 |
∗ Not all study subjects underwent sleep studies to confirm OSA.
Parameter | Obese ( n = 74) | Nonobese ( n = 62) | P |
---|---|---|---|
Hemodynamic parameters | |||
Heart rate (beats/min) | 79 ± 15 | 81 ± 17 | .66 |
Systolic blood pressure (mm Hg) | 141 ± 22 | 129 ± 19 | <.01 |
Diastolic blood pressure (mm Hg) | 79 ± 12 | 73 ± 10 | <.01 |
Echocardiographic parameters | |||
Ejection fraction (%) | 60 ± 7 | 61 ± 8 | .22 |
Pulmonary artery systolic pressure (mm Hg) | 33 ± 11 | 31 ± 13 | .55 |
Diastolic dysfunction ∗ (grade 1) | 51 (69%) | 33 (53%) | .08 |
Left atrial diameter (cm) | 3.5 ± 0.6 | 3.4 ± 0.6 | .48 |
LV diastolic dimension (cm) | 4.8 ± 0.5 | 4.5 ± 0.5 | <.01 |
Septal wall thickness (cm) | 1.1 ± 0.1 | 1 ± 0.2 | .01 |
Posterior wall thickness (cm) | 1.1 ± 0.2 | 1 ± 0.2 | <.01 |
LV mass (g) | 193 ± 57 | 155 ± 56 | <.01 |
LV mass index (g/m 2 ) | 91 ± 23 | 89 ± 30 | .72 |
Electrocardiographic parameters | |||
QRS duration (ms) | 85 ± 10 | 81 ± 8 | <.01 |
T-wave inversions | 13 (18%) | 16 (26%) | .29 |
LV hypertrophy † | 8 (11%) | 5 (8%) | .77 |
Q waves | 1 (1%) | 3 (5%) | .32 |
∗ None of the subjects had diastolic dysfunction greater than grade 1.
This study was approved by the institutional research board of Albert Einstein Medical Center.
Statistical Analysis
Data were analyzed using SPSS version 10 (SPSS, Inc., Chicago, IL). Continuous data are presented as mean ± SD. Means were compared using two-tailed Student’s t tests. Multivariate analysis was performed using the regression model. Chi-square tests were used to compare categorical variables. P values < .05 were considered significant. Coefficients of variation were used to measure interobserver and intraobserver variability in 20 random obese and nonobese subjects.
Results
The final cohort consisted of 136 subjects. Thirty subjects were excluded from the obese group and 24 from the nonobese group. Among the 30 excluded obese subjects, 17 were excluded for incomplete data and 13 for poor-quality echocardiograms. Among the 24 excluded nonobese subjects, 15 were excluded for incomplete data and 9 for poor-quality echocardiograms. In the final cohort, 74 were obese subjects and 62 were nonobese controls. The mean age was 55 ± 16 years, 32% were women, the mean QRS duration was 84 ± 9 msec, and the mean LVEF was 60 ± 8%. Of note, no subject had an LVEF < 55%, while others were more hyperdynamic. Demographic and clinical data were well matched between the two groups ( Table 1 ). Echocardiographic, hemodynamic, and electrocardiographic parameters are reported in Table 2 . Among the 72 obese subjects, 50 (68%) had BMIs of 30 to 40 kg/m 2 , and 24 (32%) had BMIs ≥ 40 kg/m 2 (morbid obesity). Interobserver and intraobserver variability was calculated for all the different VVI measurements done in the study for 20 random obese and nonobese subjects. The coefficients of variation for longitudinal, radial, and circumferential time to peak velocities were 5%, 6%, and 6%, respectively, for interobserver variability and 5%, 5%, and 6%, respectively, for intraobserver variability. The coefficients of variation for longitudinal and circumferential strain were 8% and 8%, respectively, for interobserver variability and 7% and 8%, respectively, for intraobserver variability.
VVI Analysis
Longitudinal Velocity
Among the obese subjects, 9.4% ( n = 7) had longitudinal septal–to–lateral wall time to peak delay > 75 msec, whereas none of the nonobese subjects had evidence of dyssynchrony ( P = .01; Table 3 ). There were no significant differences in the longitudinal absolute peak velocities of the basal myocardial walls (measured in the apical two-chamber and four-chamber views) between the two groups.
Parameter | Obese ( n = 74) | Nonobese ( n = 62) | P |
---|---|---|---|
Longitudinal dyssynchrony | |||
S-L delay > 75 ms | 7 (9.4%) | 0 | .01 |
A-I delay > 75 ms | 6 (8.1%) | 2 (3.2%) | .20 |
Maximum delay > 100 ms | 31 (41.8%) | 5 (8%) | <.01 |
Longitudinal strain dyssynchrony | |||
S-L delay > 75 ms | 23 (31%) | 5 (8%) | <.01 |
A-I delay > 75 ms | 20 (27%) | 10 (16.1%) | .14 |
Maximum delay > 100 ms | 43 (58.1%) | 21 (33.8%) | <.01 |
Radial dyssynchrony | |||
S-L delay > 75 ms | 58 (78.3%) | 24 (38.7%) | <.01 |
A-I delay > 75 ms | 60 (81%) | 12 (19.3%) | <.01 |
Maximum delay > 100 ms | 67 (90.5%) | 39 (62.9%) | <.01 |
Circumferential dyssynchrony | |||
A-I delay > 100 ms | 12 (16.2%) | 1 (1.6%) | <.01 |
AL-IS delay > 100 ms | 9 (12.1%) | 1 (1.6%) | .02 |
IL-AS delay >100 ms | 12 (16.2%) | 0 | <.01 |
Maximum delay >100 ms | 20 (27%) | 1 (1.6%) | <.01 |
Circumferential strain dyssynchrony | |||
A-I delay > 100 ms | 3 (4%) | 0 | .25 |
AL-IS delay > 100 ms | 8 (10.8%) | 1 (1.6%) | .03 |
IL-AS delay >100 ms | 3 (4%) | 0 | .25 |
Maximum delay >100 ms | 8 (10.8%) | 1 (1.6%) | .03 |
Radial Velocity
Among the obese subjects, 31.0 % ( n = 23) had radial septal–to–lateral wall time to peak delay > 75 msec, compared with 8.0% ( n = 5) among nonobese subjects ( P < .01). There were no significant differences in the radial absolute peak velocities of the basal myocardial walls (measured in the apical two-chamber and four-chamber views) between the two groups.
Circumferential Velocity
Among the obese subjects, 27.0% ( n = 20) had maximum opposing wall time to peak circumferential delay > 100 msec, compared with 1.6% ( n = 1) among nonobese subjects ( P < .01). There were no significant differences in the circumferential absolute peak velocities between the obese and nonobese subjects.
Strain Analysis
Longitudinal Strain
Among the obese subjects, 58.1% ( n = 43) had maximum opposing wall time to peak delay in longitudinal strain > 100 msec, compared with 33.8% ( n = 21) among nonobese subjects ( P < .01). There were no significant differences in longitudinal strain between the two groups.
Circumferential Strain
Among the obese subjects, 10.8% ( n = 8) had maximum opposing wall time to peak delay in circumferential strain > 100 msec, compared with 1.6% ( n = 1) among nonobese subjects ( P = .03). There were no significant differences in circumferential peak strain between obese and nonobese subjects.
Comparison Between Obese and Nonobese Groups
There was significantly increased time to peak delay in longitudinal, radial, and circumferential velocities and delay in time to peak longitudinal and circumferential strain in obese subjects compared with nonobese subjects ( Table 4 ). Obese subjects had higher LV diastolic dimensions, LV mass, QRS durations, and systolic and diastolic blood pressures (but all still within normal reference limits) compared with nonobese subjects. Even after adjusting for these confounding variables, in addition to age, race, gender, LV mass index, and LVEF, obese subjects had significantly increased LV dyssynchrony compared with nonobese subjects on multivariate analysis ( Table 5 ).
Parameter | Obese ( n = 74) | Nonobese ( n = 62) | P |
---|---|---|---|
Longitudinal dyssynchrony (ms) | |||
S-L delay | 31 ± 53 | 10 ± 15 | <.01 |
A-I delay | 22 ± 37 | 13 ± 22 | .07 |
Maximum delay | 109 ± 83 | 55 ± 32 | <.01 |
Longitudinal strain dyssynchrony (ms) | |||
S-L delay | 69 ± 88 | 29 ± 33 | <.01 |
A-I delay | 55 ± 62 | 34 ± 41 | .01 |
Maximum delay | 134 ± 84 | 86 ± 54 | <.01 |
Radial dyssynchrony (ms) | |||
S-L delay | 165 ± 95 | 72 ± 66 | <.01 |
A-I delay | 153 ± 96 | 50 ± 50 | <.01 |
Maximum delay | 255 ± 105 | 133 ± 78 | <.01 |
Circumferential dyssynchrony (ms) | |||
A-I delay | 55 ± 71 | 18 ± 27 | <.01 |
AL-IS delay | 59 ± 70 | 28 ± 26 | <.01 |
IL-AS delay | 61 ± 78 | 23 ± 23 | <.01 |
Maximum delay | 97 ± 95 | 39 ± 27 | <.01 |
Circumferential strain dyssynchrony (ms) | |||
A-I delay | 22 ± 42 | 16 ± 22 | .24 |
AL-IS delay | 30 ± 48 | 20 ± 31 | .16 |
IL-AS delay | 17 ± 31 | 16 ± 23 | .91 |
Maximum delay | 44 ± 53 | 29 ± 32 | .04 |
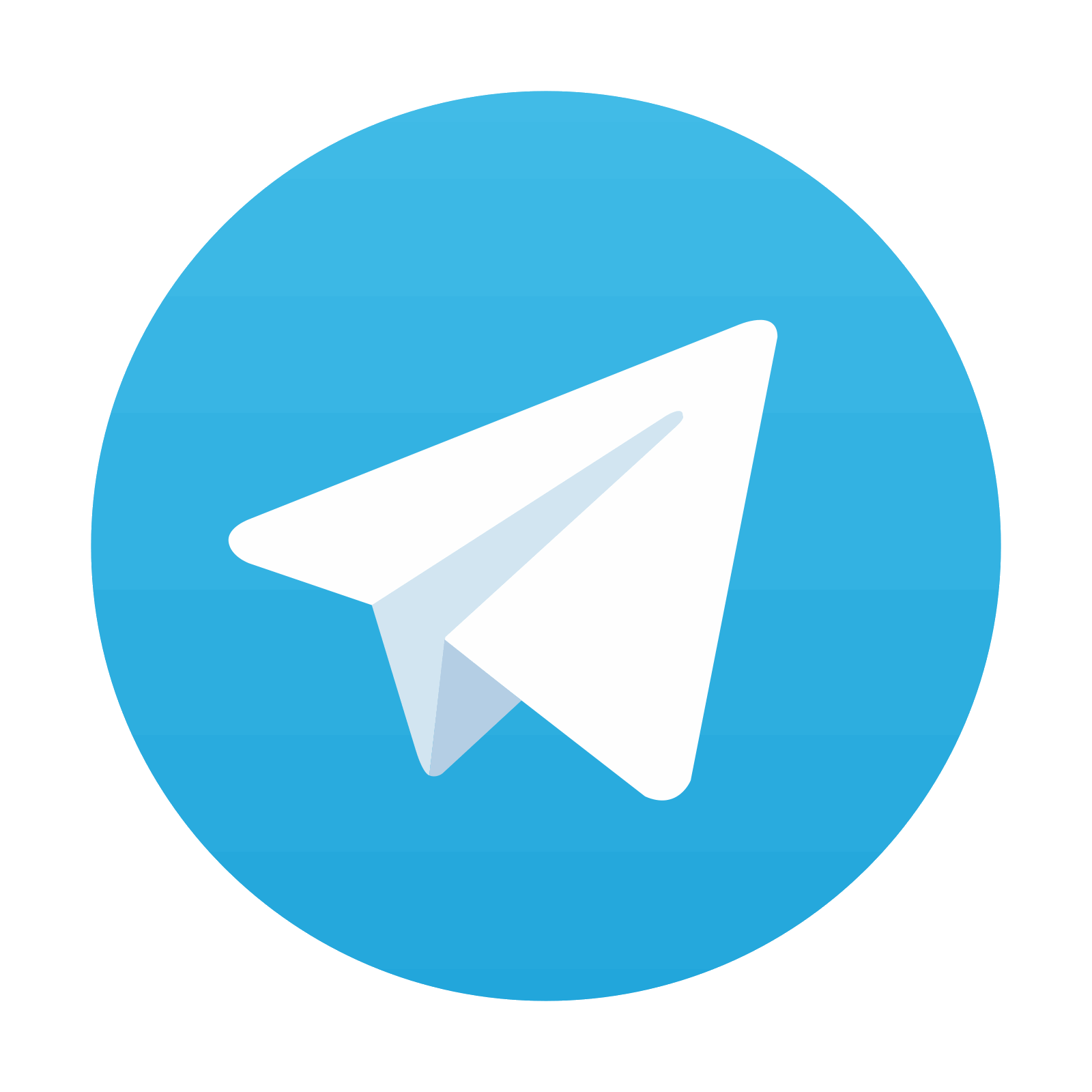
Stay updated, free articles. Join our Telegram channel
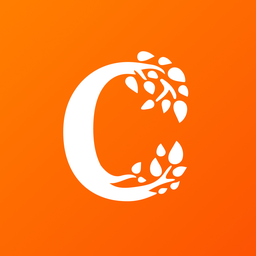
Full access? Get Clinical Tree
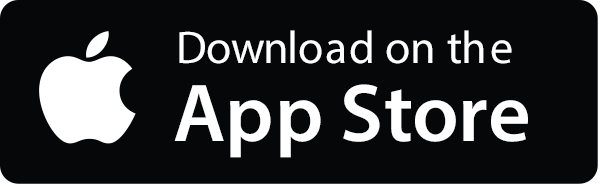
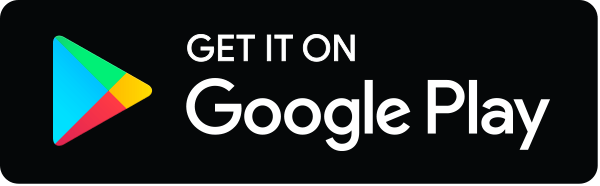
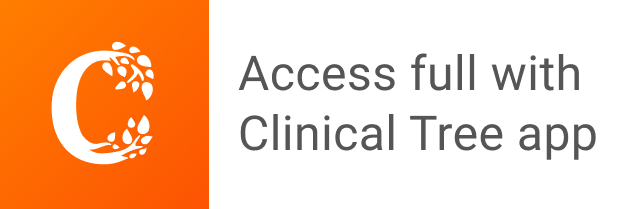