Abstract
There are many and varied devices currently approved for the administration of inhaled treatments for use in different parts of the world. Well-developed national and local guidelines and training programs for health professionals are important in ensuring that patients are prescribed the best available devices and formulations for their age and disease condition, as well as being trained adequately in their optimal use. Despite training, surveys have shown diverging practices between health care centers for using and testing efficacy of inhaled treatments. This demonstrates the confusion that arises due to the lack of consistency concerning advice from trained therapists and prescribers about the use of devices to administer inhaled treatments. This review aims to provide a summary of current inhalation devices, with advantages and caveats of each. This is done within the context of device development and how device options can be considered for appropriate treatment delivery for children with airway disease, such as asthma.
Keywords
aerosol therapy in children, respiratory drug delivery
Aerosol therapy is the cornerstone of treatment for many respiratory disorders in children. For those with asthma, the use of inhaled β-agonists and corticosteroids has formed the basis of optimal therapy for many decades, while inhaled antibiotics are increasingly being used to treat patients with cystic fibrosis and other forms of bacterial bronchitis (with or without bronchiectasis). The inhaled route has the advantage of more rapid onset of action for β-agonists; reduced systemic exposure for a given therapeutic effect for a range of drugs including corticosteroids, β-agonists, and antibiotics, and it also permits the use of certain drugs such as tobramycin, which are not readily absorbed by the enteral route. The improved therapeutic index of inhaled therapy as compared with oral therapy for the treatment of pulmonary disease is of course relative and systemic side effects do occur with high doses. For example, significant adrenal suppression can result from the use of high doses of inhaled corticosteroids (ICS), this being more likely when doses in excess of licensed doses are used.
While a great deal of research has gone into the development of devices that enable more efficient delivery of drugs to the lung, the commercial inhalers that are most widely used, particularly for children, are largely based on technologies that are more than 60 years old. These systems include pressurized metered dose inhalers (pMDIs), which may be used with or without an attached spacer or holding chamber. Few patients can use pMDIs optimally without spacers or holding chambers, which may be valved or unvalved. Spacers have the advantage of removing much of the upper airways’ deposition of drug, which helps reduce local side effects. Thus, spacers should be used by anyone prescribed an ICS in a pMDI. Dry powder inhalers (DPIs) are currently dependent on a forceful inhalation to aerosolize the drug and hence are not suitable for preschool children. However, they do represent a valuable class of delivery system both for the treatment of asthma and, increasingly, for the delivery of antibiotics. Traditional jet nebulizers, whose basic design goes back many decades, are being used less frequently while devices such as vibrating mesh nebulizers, which are quiet and provide more rapid delivery of drug, are being used increasingly for antibiotic therapy.
Advantages and Disadvantages of Aerosols for Drug Delivery
Inhaled aerosolized drugs enable more direct access to the respiratory and cardiovascular systems ( Table 16.1 ), which both include lung perivascular tissues. Aerosol drug delivery is most commonly used in children for the treatment of respiratory conditions such as asthma, cystic fibrosis, croup, and bronchopulmonary dysplasia. An inhaled entry route provides relatively unhindered access to actively exchanging capillary beds in the lungs. This ensures more rapid onset of drug action within the target organ compared with other administration methods such as oral (swallowed solid or liquid doses) and subcutaneous or intramuscular injection routes. Therefore a lower dose is needed for an equivalent effect and aerosol delivery has the advantage of reducing systemic side effects.
Advantages | Disadvantages |
---|---|
Improved therapeutic index compared with systemic administration (reduced incidence of systemic side effects for a given therapeutic effect) | The more portable metered dose devices (pressurized metered dose inhalers used alone, and dry powder inhalers) require specific inhalation techniques for optimal delivery |
More rapid onset of action for bronchodilators | Correct use of current portable devices is not intuitive; repeated training in optimal use is often essential |
Ability to administer drugs to the lungs that are not effective via the oral route due to poor gastrointestinal absorption or hepatic first pass elimination | Drug delivery is impaired in diseased regions of the lungs resulting in more central and heterogeneous distribution as lung disease progresses |
Devices used for aerosol drug delivery vary in design, which can be tailored for application or dose. However, instructions for use and optimal inhalation techniques vary greatly depending on device type, which can lead to confusion and misuse if patients are prescribed multiple devices for different drugs. Hence, to achieve optimal benefits, instructions for using a device are best achieved when given through personal professional guidance. Consistency of dosing can be an issue as this is affected by many factors including tissue and systemic absorption and airway obstruction as well as training and ability of the patient to perform the required inhalation technique. In addition, the patient’s cognitive ability to use the device and anatomical factors specific to the patient’s age or disease condition may affect the efficacy of drug delivery.
A good understanding of the principles that determine aerosol particle deposition within the lungs enables health professionals to assess the best drug-device “fit” for patients of different ages and disease conditions.
There is a wide range of types of aerosol delivery systems and formulations currently in use or in development; the focus of this chapter will be on aerosol delivery systems and therapeutic applications currently in use in children.
What Is a Therapeutic Aerosol?
Aerosols are considered to be biphasic as they contain a gaseous and a particulate phase, which in practice equates to a gas in which solid and/or liquid particles are suspended. The “gas” in which the aerosol is carried may be independently generated as part of the device itself (e.g., nebulizers using ambient air, medical air, or oxygen; pMDIs using hydrofluoroalkane [HFA] propellants). Alternatively, devices may require the patient’s inspiratory air flow to disperse and “pull” the aerosol into the lungs (e.g., most DPIs currently used). The particulate phase consists of the therapeutic agent, generally in combination with additive compounds. Solid aerosols may consist purely of the therapeutic agent (e.g., Turbuhaler) or may contain a carrier (e.g., Accuhaler). Liquid aerosols may be solutions or suspensions; in either case, the solubility of the drug (whether in saline or propellant) will affect the aerosol characteristics. In addition, for solutions, the drug concentration and viscosity may be an important factor. The aerosol generation method of the device and the drug formulation in combination will affect the type of aerosol created and the efficiency with which the aerosol deposits in the lungs.
Principles of Aerosol Delivery
In general, the higher the proportion of drug that can bypass the upper airways and deposit in the lung, the greater the therapeutic response obtained from a given label or nominal dose. Some factors that affect this therapeutic efficacy are listed in Table 16.2 ; of these, the particle size of the aerosol and the velocity at which it is generated and inhaled are two key determinants.
Device and Formulation-Related Factors | Patient-Related Factors |
---|---|
Particle size | Age |
Particle velocity | Inspiratory flow rate |
Hygroscopic properties | Breathing pattern (inspiratory volume, rate) |
Drug viscosity and surface tension | Nasal versus mouth breathing |
Suspension versus solution | Anatomy (upper and lower airways) |
Disease severity | |
Physical and cognitive ability | |
Adherence, contrivance |
Mathematical modeling and deposition studies have shown particles with a mass median aerosol diameter (MMAD) of greater than 5–10 µm are likely to be removed in the upper airways (by way of comparison a red blood cell has a diameter of ~7 µm). The proportion of particles within this range penetrating into the lower airways is in large part dependent on the inspiratory flow. The slower the inspiratory flow the greater chance particles have to follow the inspiratory flow rather than impact in the upper airways. More particles will reach the lower airways if inhalation is via the mouth rather than the nose. Particles less than 1 µm are generally exhaled as their inertia and settling velocities are very low, though particles in the nano-micron range do deposit with increasing efficiency by bypassing the upper airways and depositing through Brownian motion. (A 1 µm particle carries 1000 less drug mass than a 10 µm particle.) Within the so call “respirable range” (roughly 1–7 µm) the pattern of distribution varies with finer aerosols tending to deposit more peripherally and the coarser ones tending to deposit in the conducting airways. The pattern of deposition in an individual subject is influenced by particle size (affecting the likelihood of depositing through impaction or sedimentation, inspiratory flow (affecting the likelihood of impaction in upper and central airways) breath holding (influencing the deposition by sedimentation distally), as well as airway geometry, which can be influenced by age, natural variation, and disease.
Currently available commercial inhalers are primarily designed to generate heterodisperse or polydisperse aerosols ( Fig. 16.1 ), with a mixture of particle sizes within a given “respirable” range, to ensure deposition in the different regions of the lung and, in part, to compensation for variability in patient use of the devices. Regional differences in drug deposition within the lung affect local responses and thereby treatment efficacy.

The most important consideration with aerosolized drugs is to maximize drug deposition in the required areas of the respiratory tract. Three main factors govern this: inertial impaction, gravitational sedimentation, and diffusion. In addition, the electrostatic charge on both the aerosol and the respiratory mucosa may affect drug deposition.
Drug formulations for aerosol therapy consist of either aqueous solutions or suspensions. Solutions are a mixture of two or more components forming a homogenous molecular dispersion in a single-phase system. Suspensions consist of a dispersed system where insoluble solid particles are dispersed in a liquid medium. Colloidal suspensions are made up of solid particles less than 1 µm in diameter, whereas coarse suspensions contain solid particles greater than 1 µm in diameter. Wetting agents are usually required for the dispersion of solid particles in suspension and generally consist of surfactants, which may make up 0.05%–0.5% of the drug suspension.
Physical Principles Affecting Aerosol Delivery and Deposition
Particle Size
There are many factors that can affect the site and amount of aerosolized drug depositing in a patient’s airways (see Table 16.2 ); the aerosolized particle size is one of the key determinants. Particles less than 3 µm will deposit in the lower and smaller airways, whereas particles greater than 5 µm generally deposit in the oropharynx or upper airways. Therefore minimizing the particle size of aerosolized drugs is essential for targeted deposition in the smaller airways. Due to the proportional cubic increase in volume of drug that can be carried with unit increase in the diameter of the particles, larger particles carry a much greater volume of drug. However, aerosols that contain a large proportion of particles greater than 15 µm in diameter will deliver most of the drug available for inhalation to the oropharynx. This results in little therapeutic effect and increased systemic exposure. Measurement of particle size is complicated by variability in the shape of aerosolized droplets, which may be irregular rather than strictly spherical. For this reason, particles of different shapes that behave in a similar manner (aerodynamically) are grouped together and visualized as spherical droplets with a common diameter, known as the aerodynamic diameter and geometric standard deviation (GSD). This is defined as the diameter of a sphere of unit density with the same terminal sedimentation velocity in air as the particle or droplet in question, and is usually determined by the mass of the droplet. Most forms of aerosol therapy generate droplets of varying sizes known as polydisperse aerosol droplets, as opposed to monodisperse particles, which are characterized by uniformly sized particles of the same aerodynamic diameter. Polydisperse aerosols are further characterized by half of the aerosol mass having an aerodynamic diameter below the mass median aerodynamic diameter (MMAD), and half with diameters greater than the MMAD. Overall, this means lung deposition for a highly polydisperse aerosol with an MMAD of 3 µm would be much lower than that for a monodisperse aerosol of the same diameter, whereas the reverse would be the case if the MMAD was greater than 5 µm.
Optimizing the delivery of inhaled aerosols to patient populations is most important when drugs such as corticosteroids are being administered, especially in the pediatric age group. Ideally, drug delivery to the airways should be maximized, with minimal oropharyngeal and gastric deposition.
Inertial Impaction
The way in which a particle travels in an airstream depends on momentum, which is determined by mass and velocity. High velocity ensures continued movement in a particular direction due to inertia despite obstacles or bends. However, these may cause the direction of air flow to change as when particles approach a surface such as the back of the throat or a bifurcation of the airways. Importantly, inhaled particles may not follow the direction of air flow, and instead may impact on the surface. Therefore particles with a higher momentum, such as larger particles or those with a higher velocity are more likely to impact in the oropharynx or the larger airways. Hence inertial impaction has a greater effect on larger particles (>3 µm), and in areas where the velocity of inhaled particles is greatest; such as the oropharynx and upper airways.
Gravitational Sedimentation
All particles will settle under gravity. Particles will accelerate to a steady terminal velocity when gravitational force is balanced by the resistance of air through which it is traveling, which is aided by breath holding.
Larger particles become filtered out by the upper airways and, as the velocity of these decreases, impaction is most likely to occur in the oropharynx, meaning particles greater than 15 µm are unlikely to enter the trachea. Even for particles less than 15 µm, further deposition of the larger particles will occur at airway bifurcations.
Particles less than 3 µm are unlikely to be affected by inertial impaction in the upper airways; therefore sedimentation due to gravity is the most important mechanism for deposition of these particles in the smaller airways. Within the less than 3 µm size range, the effect of sedimentation is greatest on the larger particles (>0.5 µm) that have escaped deposition due to initial inertial impaction. Breath holding after inhalation of the aerosolized particles helps deposition in the airways due to sedimentation. The rate of deposition though sedimentation reduces exponentially over time and as a generalization there is very little benefit to be gained from breath holds of greater than 10 seconds.
Brownian Motion/Diffusion
Particles less than 0.5 µm are small enough to be perturbed by surrounding gas molecules. This bombardment (impaction with gas particles) can interrupt intended particle routes, which will move particles towards the surface of the respiratory tract, with the observed diffusivity being inversely proportional to the diameter of the particle. For particles of 0.1–1 µm, deposition by sedimentation and diffusion is very slow but below this size (which includes a number of constituents of tobacco smoke) the efficiency of deposition through the effects of Brownian motion increases significantly, which is one of the reasons there are significant concerns regarding the increasing use of nanoparticles in manufacturing processes.
Electrostatic Attraction
Deposition may occur due to attraction between charged particles in the inhaled aerosol and an induced charge on the mucosa of the respiratory tract. The extent to which this factor affects drug delivery and deposition has not been investigated in detail. The drug and other components of the formulation, device materials, and the generation of the aerosol from the device itself, can all affect the electrostatic charge of an emitted aerosol particle.
Patient-Related Variables
Quite apart from the device and drug formulation related factors, patient related variables can markedly change the effective dose of aerosolized drug received by patients (see Table 16.2 ). These factors include anatomical and physiological variation between subjects as well as disease severity and distribution. However, the most important patient variable is their ability to use the device, both effectively and consistently, which is influenced in part by the quality of training provided.
As noted above, airway obstruction due to structural disease as in progressive bronchiectasis, bronchoconstriction as in asthma, or intraluminal mucus all contribute to more central deposition with hot spots in regions of flow limitation and distal areas of little or no drug delivery. For asthma, this is of relatively little consequence as the ICS lead to progressive improvement and improved homogeneity of deposition while β-agonists given in the face of diffuse constriction when the patient is symptomatic are probably redistributed to more peripheral regions of the lungs via the bronchial circulation. In progressive disease such as CF, the effectiveness of inhaled therapy is increasingly compromised as progressively less drug reaches the more diseased areas.
Age
In some respects, the greatest challenges for using inhaled therapy result from cognitive and physical limitations at each end of the life course. For children, the period from birth to primary school is one of great change in physical and cognitive ability, which impacts their ability to use inhalers initially designed for use by adults. Conventional jet nebulizers with constant output can be used with standard tidal breathing at any age and can be used by infants and toddlers but they are relatively expensive, time consuming, inefficient, and often poorly tolerated by very young children (due to the noise and need to have a closely fitting mask). A holding chamber with a facemask can very effectively deliver drug to the lungs proving the infant/young child is not upset and will tolerate a closely fitting mask. Crying or screaming results in little or no drug reaching the lungs due to impaction in the upper airways, while if the mask is not closely fitting the patient inhales entrained air mainly rather than aerosol.
By their third birthday, most subjects are able to learn the “panting” technique with a mouthpiece, in which they take repeated inspiratory and expiratory breaths through their mouth when inhaling from a holding chamber. By 5–7 years of age, most will be able to master the optimal inhalation technique from a holding chamber with a slow inspiratory breath over 4–5 seconds followed by a 10-second breath hold or the rapid forceful inhalation required to optimally aerosolize drug from DPIs.
In terms of drug delivered, the absolute dose delivered to the lungs tends to increase over early life. For nebulized therapy, drug delivery probably reaches a maximal in the early preschool years as the inspiratory flow exceeds the flow of the nebulizer and the increased tidal volume consists of entrained air. However, drug delivery on a weight corrected basis is likely to be influenced by the device. The absolute dose delivered from a DPI with a reasonably steep dose to lung flow dependency curve may continue to increase into the teen years. However, the dose delivered per kilogram may be relatively constant for DPIs that are less flow dependent. The absolute dose delivered from a pMDI holding chamber combination may be fairly constant across age but the dose per kilogram will fall from the primary school ages as the child grows.
Regimen and Device Compliance
Compliance with an optimal treatment regimen is commonly poor in all therapeutic areas resulting in suboptimal health outcomes. That is patients frequently miss multiple doses for a variety of reasons. The situation with inhaled therapy is compounded by the need to use prescribed inhalation techniques with many studies indicating that the majority of patients, physicians, and pharmacists are unable to use devices optimally. Hence, for inhaled therapy, true adherence to a recommended treatment regime is the product of regimen compliance × device compliance (is the device used at all) × (is the device used effectively).
If the device is not taken out of the drawer the inhaler technique is irrelevant, but true adherence can still be zero if the patient attempts to take the treatment in line with the prescribed pattern of use but the patient is unable to use the device effectively. Reasons for being unable to use the device (lack of competence) include lack of adequate instruction compounded by lack of reinforcement while for others ineffectual use is due to ignoring what they know and using it efficiently (contrivance—contriving to use it inefficiently), such as the patient who demonstrates a perfect technique with a pMDI spacer but who “knows” he does not need to use the spacer and simply fires it into their mouth while taking a rapid inspiration.
The importance of true compliance can be illustrated if one considers “difficult asthma.” In general, certainly in pediatrics, there are only three causes for difficult asthma in the vast majority of patients.
- 1.
A misdiagnosis of symptoms being attributable to another condition all together, such as bacterial bronchitis.
- 2.
Asthma and a comorbidity such as a persistent bacterial bronchitis (often secondary to poor control) or an intercurrent pertussis infection.
- 3.
Ineffective drug delivery, which may be due to poor regime compliance or device compliance (due to lack of competence or contrivance).
For those who require regular therapy because of ongoing symptoms or significant exacerbations, the evidence suggests that adherence probably needs to exceed 80%—that is of a potential 14 doses a week (twice daily dosing) the patient should not miss more than 3 doses. Fewer than 20% of subjects who require this intensity of treatment appear to reach this threshold for a wide variety of reasons such as fear of side effects, concerns regarding development of “dependency,” and financial considerations. “Drug holidays” are frequently observed in clinical trials suggesting that patients are testing to determine whether medication is still required. However, the most commonly reported cause is simply forgetting to take the medication. Failure to develop a routine that deals with the need to take the medication regularly and with consistency is a common factor amongst those with poor compliance. Unfortunately, lack of explanation regarding the rational for adhering to regular medication and confusing messages from different health care professionals compound the problem. Until health care professionals are able to do the simple things well this situation is likely to persist.
Evidence suggests that competence and contrivance can both be addressed with effective education by healthcare professionals, but these messages need to be repeated and reinforced. Education has, in general, little or no impact on behavior in the vast majority of patients unless considerable resources are invested and to date, the most effective interventions appear to be monitoring with feedback. Studies that provide patients with feedback regarding their behavior have consistently shown improved adherence and studies that are more recent have started to demonstrate clinically important improvements resulting from the improved adherence. It seems likely that more devices will have data loggers incorporated or will be provided with an add-on data logger that can provide real time feedback. Improved data provides for more open and honest discussion between physician and patient.
Assessment Techniques
Aerosol drug formulations and devices need to be assessed in combination because the formulation can greatly affect the type of aerosol emitted from a given device. These evaluations may be purely in vitro assessments of aerosol generation and efficiency of aerosol delivery, or in vivo measurements of drug delivery to the patient and deposition within the lungs. In general, these aerosol-specific assessments are conducted as part of the development and initial assessment of the performance of an inhaler, prior to conducting larger scale trials of clinical outcomes in different patient groups.
Aerosol Generation
Assessment of aerosol characteristics with in vitro techniques consists of the measurement of those characteristics of the aerosol that have a major effect on drug delivery to the lungs. The efficacy of drug delivery from the aerosol generated by a particular device is largely dependent on two factors:
- 1.
The range of particle sizes present in the aerosol cloud, termed the particle size distribution .
- 2.
The total output of drug.
The size distribution of the particles or droplets making up a therapeutic aerosol can be described in three ways: the frequency of occurrence of particle volumes, masses, or counts. The description of the aerosol cloud can then be expressed as, respectively, the volume median diameter (VMD), the mass median diameter (MMD), and the count median diameter (CMD). These diameters are those above and below which, respectively, half the volume of the aerosol, half the mass of the aerosol, and half the number of particles in the aerosol reside. The VMD and the MMD will be identical if all the particles comprising the aerosol have the same density, as is normally the case when a drug in solution is nebulized. As the amount of drug contained within a droplet or particle is defined by the volume of the particle, and this is related to the cube of the radius of the particle, the CMD is not useful in assessing the likelihood of successful drug delivery from a therapeutic aerosol, as one droplet 5 µm in diameter will contain 1000 times as much drug as one droplet 0.5 µm in diameter and therapeutic aerosols are generally polydisperse, consisting of particles with a range of diameters. The particles may also be irregular in shape and differ in density, which will alter their behavior when entrained in an air flow. The aerodynamic behavior of a particle is best described by its aerodynamic diameter, that is, the diameter of a spherical particle of unit density that behaves in the same way when entrained in a defined air flow. Measurement of the MMAD, of an aerosol by inertial impaction, helps to describe and predict the behavior in an air flow of a polydisperse aerosol consisting of particles of different shapes and densities. The extent to which the aerosol is polydisperse is defined by the GSD (σ g ), the ratio of the particle diameter below which 84.3% of the mass is contained to that below which 50% is contained. The larger the GSD, the more polydisperse the aerosol. Most inhaler devices produce aerosols consisting of large numbers of small particles with progressively fewer larger particles, within the range of approximately 1–10 µm MAD.
All of these factors can be greatly affected by the way in which the inhaler is stored and used. Optimizing the particle size distribution and output from an inhaler by careful formulation, and good storage and use practice, will maximize the amount of drug available for inhalation by the patient.
Particle Size Distribution
There are two main methods of assessing the particle size distribution from a given aerosol: inertial impaction and laser diffraction. Inertial impaction measures the actual drug content within particles of a precalibrated size range while laser diffraction measures particle size by volume. For most drug solutions and for dry powders consisting only of the drug compound, we may assume that the drug is evenly distributed through the aerosol droplet or particle.
Inertial Impaction.
The generated aerosol is drawn by an entraining air flow (defined and calibrated for each device) through the impaction device, which consists of a series of stages. Each stage acts as a low pass filter, where “larger” particles, above the defined aerodynamic diameter cut-off for that stage, impact and are retained, while “smaller” particles remain entrained in the air flow to move further through the device. At each consecutive stage, remaining particles above the cut-off aerodynamic diameter of that stage are retained, while particles of smaller aerodynamic diameter remain entrained until they reach the final stage, which consists of an absolute filter to remove very small particles that have not impacted further up the series of stages.
There is a wide variety of impaction devices available. Cascade impactors collect particles in discrete size ranges that impact on a series of solid stages, sometimes coated with grease to trap the impacting particles and prevent them from bouncing off the stage, to be reentrained and drawn on to a lower stage. Liquid impingers have a layer of solvent on each stage (except the absolute filter), which performs the same function. Each impaction device may be made up of a different number of stages. The greater the number of stages, the greater is the resolution in the characterization of the particle size distribution of a particular aerosol. The particle size cut-offs for the stages in each impaction device are calibrated for a particular entraining air flow, which may also affect the characteristics of the aerosol. Particles reaching the lower stages of inertial impactors (with particle size ranges of around 1–5 µm) are often referred to as “respirable” particles, though the amounts of drug captured on these stages tend to be a significant overestimate of the proportion of drug emitted from the inhalation device that would reach the lungs of patients. An example of two impaction devices, a multistage cascade impactor and the Next Generation Impactor (Copley, UK), are shown in Fig. 16.2 .

Once the aerosol dose has been drawn through the device, the air flow is stopped and the surface of each stage is washed separately to remove impacted particles. The amount of drug retained on each stage is assayed, usually using high performance liquid chromatography or ultraviolet spectrophotometry. The sum of the amount of drug deposited on these stages provides the total drug output of the device tested. The components of the inhalation system, such as the pMDI actuator or the nebulizer body, may also be washed to measure the amount of drug retained by the device and not administered to the patient. Using this method, an assessment can be made of the amount of drug contained within each fraction of the total range of particle sizes contained within the generated aerosol, and hence a prediction can be made of the amount of drug that will reach the target area within the lungs. Any changes in particle size distribution and total drug output in the aerosol generated by an inhaler under different conditions, or with changes in formulation can also be assessed.
The particle size distribution is given in discrete ranges, not the continuous spectrum of sizes actually generated by the device being tested, which is a disadvantage of inertial impaction techniques. Hence, direct comparisons between different impaction devices are not easy, especially if they are calibrated for different entraining air flows.
Laser Diffraction.
Measurement of particle sizes using laser diffraction involves passing the aerosol through a laser beam, resulting in light scattering (Fraunhofer diffraction) by the edges of the aerosolized particles. The scattered light passes through a lens that focuses the rays onto a series of detectors. The angle of diffraction is inversely proportional to the droplet size. This information is used to calculate the numbers of aerosolized particles at any volume in the aerosol. This method only measures particle volumes (VMD) and not the actual amount of drug present. For aerosols generated from a solution of a drug, as produced by a nebulizer, the total volume of the combined droplets of any given size is proportional to the amount of drug contained within those droplets, and so may be useful in predicting therapeutic efficiency. For aerosols where the drug is contained within particles suspended in the aerosol, the particles may be larger than the smallest droplets, and so droplets in this range would contain no drug.
Measurement of particle size distributions using either of the techniques described above is complicated by a number of factors. Particle size distributions can vary greatly with the conditions of testing, such as inhalation flows through impaction devices, and driving gas flows through nebulizers. Changes in particle sizes once an aerosol has been generated can also occur due to factors such as hygroscopic growth, which is dependent on humidity and the composition of the aerosolized particles, and evaporation of the more volatile components of a drug suspension.
Total Drug Output
The total amount of drug contained in a dose of aerosol generated by an inhalation system can be measured by delivering the aerosol through a low resistance filter, and assaying for the amount of drug deposited on the filter. Another method is measurement of the weight of the inhalation device before and after aerosol generation, but this method usually greatly overestimates aerosol output due to high levels of evaporation. Total drug output, as well as the particle size distribution, can be determined using impaction devices, if the masses of drug in each size fraction are added together. This is not possible using laser diffraction techniques.
Factors other than particle size and output can also play an important role in the assessment of an inhalation system. In the case of nebulizers, the time taken to deliver the aerosolized drug is also important, especially in the pediatric age range. Factors such as driving gas flows through the nebulizer or inhalational flows through pMDIs, spacers, and DPIs can significantly affect all the parameters mentioned above.
Delivery
In vitro studies under carefully controlled laboratory conditions are used to assess the aerosol generated by a particular device, giving relatively reproducible results. While these techniques provide useful information on optimizing aerosol generation, drug delivery from these same aerosol generation devices, to patients in a clinical situation, can still be extremely variable, largely due to variation in the patient’s inhalation technique.
Filter studies can be used to assess aerosolized drug delivery to patients using different inhalation systems and inhalation techniques. For these studies, a low resistance filter is placed between the inhaler and the patient’s mouth. When the device is actuated and the patient inhales, the amount of drug that would normally be inhaled by the patient is deposited on the filter. This equates to the “total body dose” that would have been administered to the patient. The filter can then be washed, and the amount of drug deposited can be assayed. This method is noninvasive, since the patients do not inhale any drug, and the performance of the inhaler can be assessed by measuring the “total body dose” delivered to the patient over a range of patients’ breathing patterns. The approach provides some idea of the variability that inevitably occurs in the clinical use of these inhalers especially in children. It does not however provide any information on the particle size distribution of the delivered aerosol, which can be influenced by inspiratory flow when using a nebulizer or dry powder inhaler, and gives no information about the pattern of deposition of the drug in the lung, mouth, throat, esophagus, and stomach that would have occurred if the filter was not present. To obtain information about where the inhaled drug deposits in the body, deposition scintigraphy by imaging following inhalation of a radioisotope labeled aerosol may be utilized.
In vivo Deposition
Deposition scintigraphy is an imaging modality, which enables the investigator to visualize the in vivo distribution of drug within the patient’s body. Two-dimensional (2-D) planar scintigraphy, three-dimensional (3-D) single photon emission computed tomography (SPECT), and positron emission tomography (PET) may all be utilized ; however planar scintigraphy involves the lowest radiation exposure and is generally the method utilized in children. The data obtained from scintigraphy studies takes into account both the drug-device delivery characteristics and the effect of the patients’ inhalation techniques in determining the amount and distribution of aerosolized drug depositing within the body. The drug is labeled with a low level of a radioisotope and gamma scintigraphy is used to quantify the deposition of the label in the lungs after inhalation by the patient. These tests are necessary to ensure that the aerosolized drug produced by an inhaler is reaching the target site, and the number of such studies should be minimized by only performing them with optimal delivery systems. Hence, initial assessments of any new inhaler should be carried out using in vitro and filter studies.
The most common radioisotope used for aerosol deposition studies is technetium (Tc 99m ), a gamma emitter that has a short half-life of 6 hours. The half-life in the lungs is much shorter than this, about 15–20 minutes, as the isotope is rapidly cleared from the lungs. Mouth and throat deposition can be reduced by the use of a spacer with radiolabeled pMDIs, as well as mouth washing and gargling after inhalation of the radiolabeled aerosol.
Useful results can be obtained from images obtained after administration of a very low amount of activity (for planar scintigraphy) ( Fig. 16.3 ), comparable to the radiation exposure received during a 12 hour plane flight, or from two to four weeks of exposure to natural background radiation.
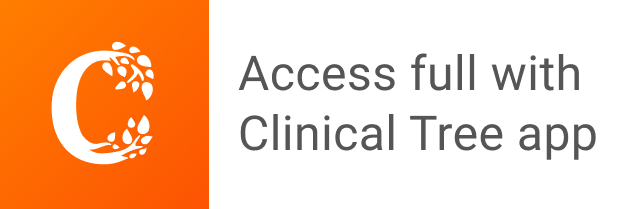