Fig. 1
Neural and nonneural pathways that contribute to airway reactivity. AVPNs airway-related vagal preganglionic neurons, ASM airway smooth muscle
Muscarinic receptors mediate the responsiveness of airway smooth muscle to acetylcholine during early development and adult life. Studies in the developing airways and porcine lung from birth to adulthood reveal maturational changes in muscarinic receptor subtypes (M1, M2, M3) that may explain pharmacologic changes during development [12]. These muscarinic receptor subtypes, coupled to the family of G proteins, mediate airway contractile responses and their modulation, although there are considerable interspecies differences in their roles. M1 receptors are largely present on neuronal tissue and ganglia and enhance contractile response to vagal stimulation in newborn animals [7, 13]. M2 receptors are located on prejunctional postganglionic cholinergic fibers in airway smooth muscle in some species and exhibit an autoinhibitory action whereby the quantal release of Ach in response to nerve stimulation is reduced due to feedback inhibition. M3 receptors are present on smooth muscle and mucus glands and airway epithelial cells, where they initiate the events leading to smooth muscle contraction, airway narrowing, and mucus secretion. In the newborn, the density of M3 receptors has been reported to be similar to that in the adult; however, they do not appear to be tightly coupled to G-protein signal transduction mechanisms that lead to smooth muscle contraction [7]. Among the many unanswered questions in the newborn are the extent of receptor subtype differentiation in human neonates, investigation of G-protein signal transduction, the role of M2 autoinhibitory receptors in modulating airway tone, and the possible effect of inflammatory lung injury on muscarinic receptor regulation and its pharmacologic manipulation.
Sympathetic Control of the Lower Airways
Extrinsic sympathetic innervation of airway smooth muscle is highly species specific, and in airway smooth muscle in humans, direct sympathetic innervation appears to be lacking. Nevertheless, circulating catecholamines activate airway adrenoreceptors to exert specific actions that affect smooth muscle contractile function. Beta2-adrenergic responses in airway smooth muscle are composed of two inhibitory actions: first, relaxation of airway smooth muscle mediated by airway beta2-receptors that are coupled to the stimulatory G-protein (Gs) and adenylate cyclase and second, inhibition of ACh release from postganglionic vagal axons through prejunctional alpha2-adrenergic and beta1 receptors in some species. Activation of beta-adrenergic receptors is the pharmacologic basis for neonatal bronchodilator therapy. Maturational studies have demonstrated that beta-adrenergic receptors in lung tissue increase with advancing gestation and subsequent postnatal development, but this may be more important for their role in surfactant synthesis and release. The airway relaxant response to beta-adrenoreceptor stimulation has been reported to decrease with advancing maturation, and several mechanisms including greater muscarinic antagonism of beta-receptor responses and attenuated expression of M2 muscarinic receptors have been proposed [14]. A potential role for adrenergic receptors in the control of airway smooth muscle in newborn infants with bronchopulmonary dysplasia is supported by observations in preterm infants with chronic lung disease in whom ophthalmic application of the alpha1-adrenergic agonist phenylephrine resulted in an increase in total pulmonary resistance and a decrease in compliance [15]. The deterioration in lung mechanics was attributed to alpha1-receptor-mediated activation of airway smooth muscle contraction.
Nonadrenergic Noncholinergic Control of the Lower Airways
Rather than representing a separate pathway for modulation of airway caliber, the nonadrenergic noncholinergic (NANC) system comprises both inhibitory and excitatory components modulated by several neurotransmitters located in intrinsic ganglia and their fibers. Reference to noncholinergic (i.e., NANC) innervations reflects the neurotransmitters known not to mediate cholinergic or adrenergic effects. The system is highly species-specific but has been identified in human airways. The NANC inhibitory component of vagal innervations is demonstrated in vivo and in vitro by measuring the response to vagal stimulation or to electrical field stimulation, after muscarinic and beta-adrenergic effects on airway smooth muscle are blocked by atropine and propranolol; then airway tone is elevated by an infusion of contractile agonist (e.g., serotonin or histamine). Under these conditions, stimulation of vagal preganglionic axons causes bronchodilation. Vasoactive intestinal peptide was initially proposed as the primary neurotransmitter of the NANC system. Subsequently, nitric oxide was also shown to act as a NANC neurotransmitter, suggesting involvement of multiple substances and molecules in airway smooth muscle relaxation with considerable interspecies variation [16].
Limited information is available about the ontogeny of this system in the airways. Activation of vagal preganglionic axons results in a NANC-mediated bronchodilation in newborn feline airways, a response that is eliminated by ganglionic blockade with hexamethonium, confirming the efferent nature of the response [17]. Comparison with the mature response is confounded by the necessity of infusing an agonist to measure a response, and the possibility that neonatal and adult airways may possess different sensitivities to the contractile agonist. NANC inhibitory innervation also appears to be functional in young guinea pigs and rat pups. In some species, NANC inhibitory responses undergo significant developmental changes; for example, NANC relaxation responses are not present in rabbits until 2 weeks of age [18]. Interestingly, allergen sensitization significantly reduced the NANC response at 2, 4, and 12 weeks of age [18], suggesting that host or environmental factors may alter the maturation of the inhibitory NANC system and predispose to airway reactivity. The NANC inhibitory system has not been adequately explored in human neonatal airways.
NANC excitatory mechanisms also play a role in modulating airway smooth muscle. Within this system, the tachykinin peptides, such as substance P and neurokinin A, have undergone some study during early postnatal development. These tachykinins are synthesized in sensory neurons and transported to sensory nerve endings from which they are released and have the ability to elicit airway contractile responses by several interrelated mechanisms. Tachykinin release from afferent C-fiber nerve endings in the airway may directly or reflexly elicit smooth muscle contraction, modulate cholinergic responses through muscarinic receptors, and induce histamine release from mast cells. In young rabbits, substance P-induced modulation of ACh release increases with advancing postnatal age [19], and in newborn piglets, exogenously administered substance P elicits weak contractile responses of tracheal smooth muscle when compared with older animals [20].
There is some debate about whether increased expression of substance P or other neuropeptides contributes to lung or airway pathophysiology. In mature animal models, chronic exposure to irritant gas increases substance P content; however, it is controversial whether this serves to aggravate airway hyperactivity or serves a protective role for airway and lung structures. Furthermore, in addition to elicit airway smooth muscle constriction, substance P may induce relaxation of preconstricted neonatal tracheal tissue by release of NO and relaxant prostaglandins [21]. Newborn and 3-week-old rats exposed to hyperoxia exhibit increased tachykinin precursor expression, increased substance P content in the lung, and increased cholinergic responsiveness, as discussed later, although the relationship of the latter to the increased substance P content is not clear [22]. Ongoing developmental studies should focus on these neuropeptide-mediated signaling pathways that modulate airway smooth muscle contractile and relaxant responses in health and disease.
Contributors to Abnormal Airway Function
Hyperoxic Exposure
The airways of preterm infants are frequently exposed to increased supplemental oxygen. This is a likely predisposing factor to the development of airway injury (Fig. 2). In newborn animal models, most notably the guinea pig and rat pup, hyperoxic exposure has been associated with development of airway hyperreactivity [22–24]. Although hyperoxic exposure may increase smooth muscle area, this effect is variable and does not, of itself, explain the development of hyperoxia-induced airway hyperresponsiveness [25].


Fig. 2
Proposed mechanisms whereby neonatal hyperoxic exposure predisposes to wheezing disorders in former preterm infants. ASM airway smooth muscle
Many factors may contribute to the increased airway reactivity that is seen after neonatal hyperoxic exposure. Therefore, studies have focused on neonatal rodent models exposed to only moderate (e.g., 40–60 %) hyperoxic exposure as this more closely simulates the clinical condition. Recent data demonstrate that 40 % oxygen exposure elicited a greater increase in airway reactivity than 70 % oxygen exposure, associated with greater airway smooth muscle thickness [26]. This might be attributed to a dominant proliferative effect of 40 % oxygen on airway smooth muscle versus a predominantly apoptotic effect at the higher oxygen level [27, 28]. An intriguing recent observation is that in a neonatal mouse model, the increase in airway reactivity after 40 % oxygen exposure during the first week was delayed until the pups were 3 weeks old [29]. These studies employed an in vitro living lung slice preparation. Alveolar morphology was comparably diminished at 1 and 3 weeks, suggesting a selective and delayed effect of modest hyperoxic exposure on the trajectory of airway smooth muscle structure or function. These findings are consistent with the clinical observation that bronchodilator therapy is of limited use in the neonatal intensive care unit (NICU) at a time when hyperoxia-induced airway smooth proliferation has not been achieved [30]. It is also possible that the benefits of bronchodilator therapy may not be sustained in the neonatal period as evident in the hyperoxia-exposed neonatal mouse model. In a model of chronic lung disease, hyperoxia-exposed newborn mice displayed decreased lung beta2-adrenergic receptor expression and were at risk for further down regulation with repeated agonist stimulation, resulting in increased airway hypersensitivity and attenuation of rescue bronchodilation [31].
Lung parenchymal injury is a well-recognized complication of neonatal intensive care and is characterized by the development of enlarged, simplified alveolar structures. This may result in decreased tethering of intraparenchymal airways; the accompanying decrease in airway lumen would increase baseline airway resistance [11]. A related observation is that lung parenchymal injury elicited by hyperoxic exposure is associated with fewer bronchiolar-alveolar attachments in a rodent model of neonatal lung injury [32]. Smaller diameter, untethered airways may well contribute to the wheezing disorders former preterm infants experience; however, altered airway smooth muscle properties are likely contributors.
Epithelial injury with loss of airway relaxant factors may also contribute to the hyperoxia-induced increase in airway contractile responses. This is supported by data from tracheal strips in preterm sheep, in which epithelium removal was associated with greater cholinergic responsiveness [33]. A similar phenomenon is observed in rat pups in which the response of lung resistance induced by vagal stimulation was increased after nonspecific blockade of NOS in normoxic animals. However, after hyperoxic exposure, NOS blockade no longer affected the contractile response induced by vagal stimulation [34]. These findings indicate that NO, released by stimulation of vagal preganglionic fibers, modulates bronchopulmonary contractile responses to endogenously released ACh in rat pups. This effect appears to be lost after prolonged hyperoxic exposure and may contribute to airway hyperreactivity under these conditions. Impairment of the prostaglandin/cyclic adenosine monophosphate (cAMP) signaling pathway may also contribute to hyperoxia-induced airway hyperreactivity [35]. These findings are somewhat analogous to observations made in young ferrets infected with human respiratory syncytial virus (RSV) in which nonadrenergic noncholinergic inhibitory responses of tracheal smooth muscle were significantly decreased for a prolonged period [36]. Of course, it is important to recognize that these animal data may not translate to the human condition.
The neurotrophins belong to a protein family essential for vertebrate nervous system development. They are also expressed in epithelial smooth muscle and immune components of the lung [37]. The neurotrophin family includes brain-derived neurotrophic factor (BDNF), nerve growth factor (NGF), neurotrophins 3, and neurotrophin 4. They share common structural features and act through their corresponding high-affinity tyrosine kinase (Trk) receptor subtypes. The rapid excitatory activity of BDNF is via activation of the TrkB receptor. BDNF appears to stabilize excitatory (e.g., cholinergic) postsynaptic receptors and/or enhance quantal excitatory neurotransmitter release probably via postsynaptic calcium signaling. During development, regulation of the lower airway appears to be influenced by neurotrophins. Data show the presence of neurotrophins and their corresponding receptor on airway smooth muscle cells in developing rat pups and upregulation of the BDNF system after hyperoxic exposure [38, 39]. Consistent with these findings, neurotrophins are overexpressed in the lower airways of RSV-infected human infants known to be at great risk for increased airway reactivity [40].
Airway smooth muscle contraction is elicited when phosphorylation of the 20-kDa regulatory light chain of myosin (MLC20) allows crossbridge formation of actin and myosin. This process of phosphorylation is regulated by calcium/calmodulin-dependent myosin light chain kinase (MLCK) isoforms. Conversely, MLC20 dephosphorylation by myosin phosphatase (MYPT) leads to relaxation. Studies in rat pups have demonstrated that hyperoxic exposure inhibited MYPT, and the resultant prolongation of MLC20 phosphorylation may have contributed to hyperoxia-induced enhanced airway contractility [41]. Both plasma membrane calcium influx mechanisms, as well as intracellular calcium release and reuptake, are involved in the contractile responses of airway smooth muscle to agonist. Even in the first trimester, immature airway smooth muscle contains many of the calcium-regulating mechanisms that are present in adult tissue, contributing to spontaneous and acetylcholine-induced calcium oscillations [42]. However, there is currently limited information on which of the calcium regulatory mechanisms are involved in mediating bronchoconstriction in the normal and injured developing airway. In this regard, in human fetal airway smooth muscle, many calcium influx pathways appear to be present, as are intracellular calcium release pathways. However, compared to adult airway smooth muscle, calcium responses to agonist appear to be smaller and slower in developing fetal airway smooth muscle cells, likely reflecting differences in the kinetics of regulatory mechanisms [28]. Importantly, exposure of fetal airway smooth muscle cells to even moderate levels of hyperoxia causes enhanced calcium responses to agonist, and this may contribute to the increased airway reactivity observed in vivo following hyperoxia.
Pressure Effects
The high deformability or compliance of the trachea in the preterm period appears to be a consequence of decreased airway smooth muscle contractility and diminished cartilaginous support. The obvious result is that tracheas, and possibly lower airways, are vulnerable to deformation during positive pressure ventilation. Greater understanding of the detrimental effects of positive pressure ventilation at high inflating pressures has decreased the risk of deformation injury to the immature airway by limiting the use of excessive ventilatory pressures. Data in rat pups indicate that enhancement of airway reactivity occurs even after short-term mechanical ventilation [43]. Abnormal mechanical stress and its effect on smooth muscle contractility serve as a useful model for characterizing one aspect of neonatal lung and airway injury [44]. Cultured airway smooth muscle cells exposed to such stress eliminate the confounding effects of deformational strain on surrounding tissues. Such studies have demonstrated strain-induced increases in cell myosin light-chain kinase (MLCK) accompanied by increased phosphorylation of the myosin light chain, all key steps in the smooth muscle contractile response [45].
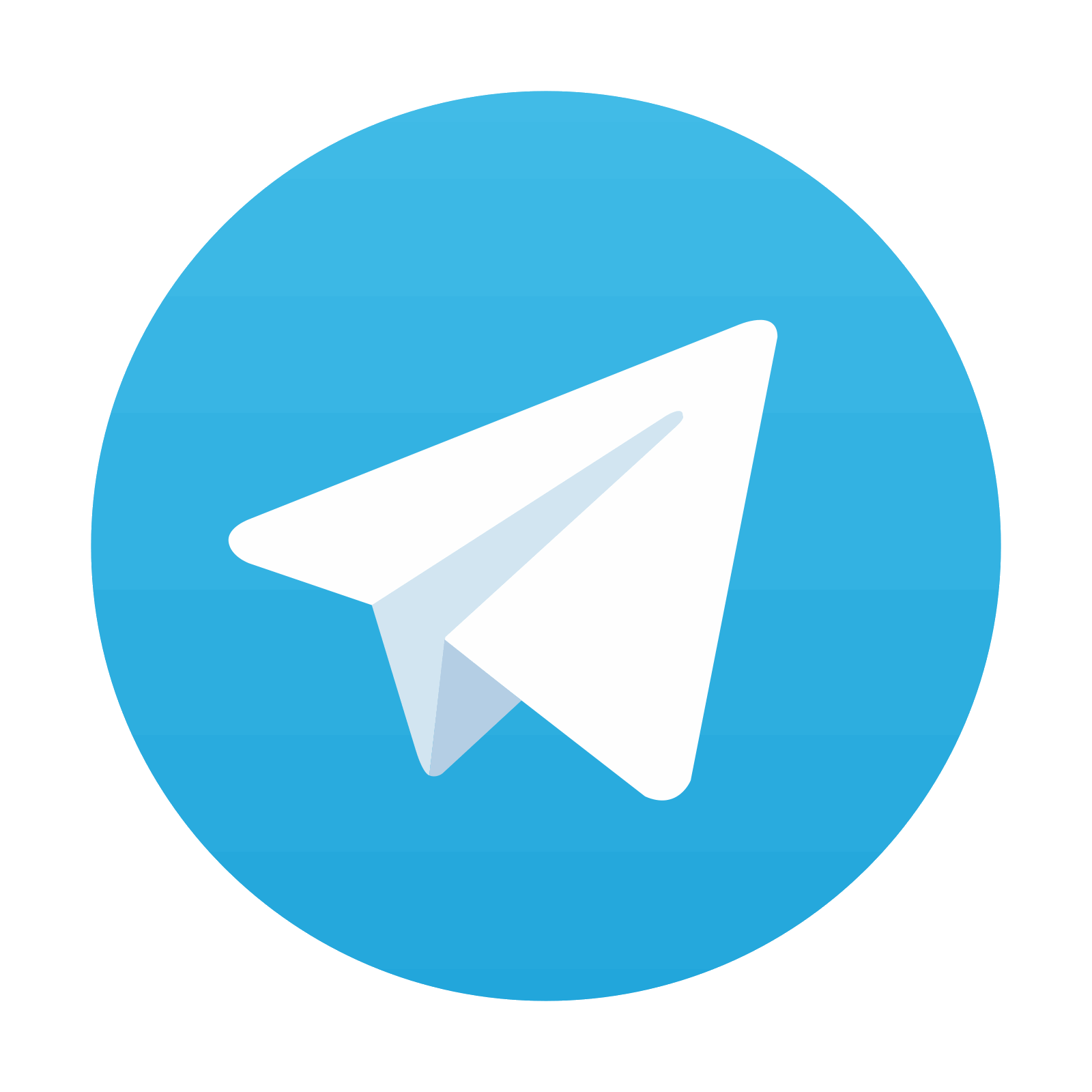
Stay updated, free articles. Join our Telegram channel
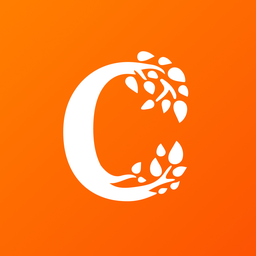
Full access? Get Clinical Tree
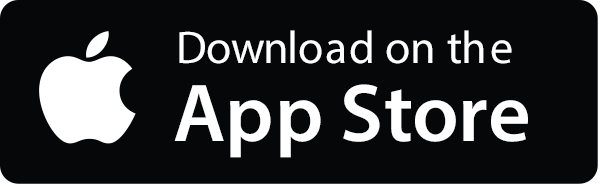
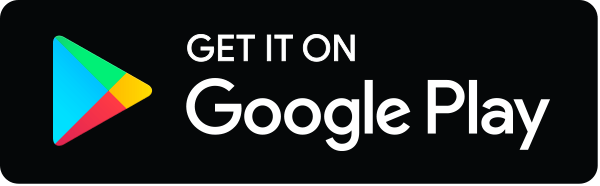