Fig. 2.1
Imported image from a 3D echocardiography dataset for 3D printing performed for evaluation of an atrial septal defect

Fig. 2.2
The atrial septum was segmented using Materialise Mimics® Innovation Suite

Fig. 2.3
Mimics® Innovation Suite was used to reconstruct a 3D rendering of the atrial septum, which is shown with the visible atrial septal defect

Fig. 2.4
Materialise Heart Print® Flex 3D printed model of an atrial septal defect from 3D echocardiography
A 3D printed heart model may be used to teach patients and their family members about the congenital heart defect and plan for repair. Currently, a 2D representation, such as a drawing on a piece of paper or whiteboard, is used to explain the procedure to patients and their family members. The visual and tactile feedback provided by 3D printed heart models markedly improves the understanding of complex structural heart defects and may be beneficial to teach medical students, residents, nurses, and other medical professionals about specific congenital heart defects [1, 6–9, 11–16]. The 3D printed heart model is expected to enhance professional training, enable practicing procedures before performing them, and help design precise prostheses prior to an interventional or surgical procedure. In complex anatomical repairs where expert opinion is required, the 3D virtual and printed models can be shared rather than inconveniencing the patient to travel long distances.
The cost and time needed to create 3D printed models may vary widely depending on the complexity of the lesion and quality of the material used for printing. As a result, it is important to consider the indications or degree of complexity of congenital heart defects for 3D printing to maximize its utility and reduce commercial misuse [9].
Patient Selection and Image Acquisition
The field of congenital heart disease has undergone major treatment improvisations over the last 4 decades. For example, the arterial switch operation has been the treatment of choice for transposition of the great arteries for the past 30 years [17]. This congenital defect was previously managed by the Mustard or Senning procedure, which could functionally correct the altered hemodynamics. The Fontan operation as the final procedure in the common single ventricle pathway has also undergone major revisions in the past 40 years [18]. However, these patients who underwent palliative procedures in the past are now presenting with cardiac complications and require advanced imaging to help form a complete picture of their clinical status. It may be difficult for cardiologists and surgeons new to the field, or who are not trained in imaging, to interpret the cardiovascular images obtained by conventional modalities in these patients. 3D printing of such complex repaired defects facilitates the understanding of the anatomical substrate. 3D printed models help in planning the appropriate interventions well in advance, which can improve the interventionalists’ or the surgeons’ preparation for the procedure. The utility of 3D printing in planning catheter intervention in pulmonary venous baffle obstruction in Mustard repair has recently been demonstrated [11]. The size of devices, size, and shape of conduits or patches, and the accessory equipment required during the intervention can also be planned, contributing to the procedure going smoothly. This may reduce procedure time and risk of radiation exposure and aid in the prevention of inadvertent complications. Some of the specific congenital heart defects for which 3D printing can make significant differences in the management are described below.
Determining the morphology of the superior and inferior bridging leaflets as well as identifying any imbalance of the valve opening into the ventricles is critical in determining the suitability for biventricular repair in atrioventricular septal defects (AVSD) [19]. Ventricular size can be underestimated due to foreshortening on conventional imaging modalities. Visualization of AVSDs by hybrid 3D printing can provide insight into the actual ventricular volumes, the relationship of the bridging leaflets to the ventricles, presence of straddling leaflets, and associated anomalies. The size of the patch required and strategies to repair the left-sided cleft valve to prevent later regurgitation can also be planned [20]. 3D hybrid segmentation and printing is especially relevant in this setting given that valvular structures are best re-created using 3D echocardiographic images.
Double-chambered right ventricle (RV) is another congenital heart defect for which 3D printing may be useful. It occurs due to muscle bundles separating the RV inlet and outlet (pulmonary artery) from the body of the RV [21]. This malformation is found in up to 10% of patients with ventricular septal defects (VSD) on long-term follow-up. The RV is difficult to image and quantify because it does not conform to the geometric assumptions made for the left ventricle. The cardiothoracic surgeon requires vital information regarding how much extra volume may be added to the RV once the muscle bundles are resected, especially in patients with corrected complex congenital heart disease or 1½ ventricular repair. The tangibility offered by 3D printed models provides the surgeon with a hands-on experience of the actual muscle resection prior to the procedure. This can have far-reaching implications such as choosing between 1½ ventricular repair versus biventricular repair (Fig. 2.5a–e) [22].


Fig. 2.5
a A 4-chamber view from CMR showing muscle bundles in the mid-right ventricle in a patient with pulmonary atresia intact ventricular septum palliated with a bidirectional Glenn anastomosis. b A 3D TEE showing the tricuspid valve. c A 3D rendering of the integrated CMR and 3D TEE datasets. d A hybrid 3D printed model showing the LV and the obstructing muscle bundles in the RV. e A hybrid 3D printed model corresponding to an echocardiographic apical 4-chamber view
Corrective surgery in double outlet right ventricle (DORV) may involve baffling of the VSD to the aorta or performing an arterial switch operation. One of the factors that influence the approach is the proximity of the ventricular septal margin to the aorta. However, DORV with subpulmonary VSD (Taussig-Bing anomaly) requires baffling of the VSD to the pulmonary artery followed by an arterial switch procedure. Commitment of the VSD to one of the great vessels (pulmonary artery or aorta) is mandatory for successful biventricular repair. 3D printed models of the heart provide accurate visualization of the relationship of the VSD to the outflow tracts so that treatment decisions regarding routability can be made [23].
Considering the complications and late failure of the Fontan procedure, Fontan conversion or takedown may be considered in some cases [24]. Hybrid 3D printed models of these complex hearts provide excellent representation of the size and relationship of the chambers and the valvular anatomy. There are recent reports of the utility of 3D printed models of the RV outflow tract in the accurate selection of patients for pulmonary valve implantation [25]. It is critical to evaluate the size and orientation of the outflow tract and possibly test out the surgical intervention on a 3D printed model prior to undertaking such complex interventions. 3D printed models of the heart and the great vessels have been found to be useful in preoperative and pre-interventional planning of stent sizes in coarctation of aorta, branch pulmonary artery stenosis, and caval valve implantation techniques [16, 26]. Custom-sized patent ductus arteriosus stents in hybrid procedures for hypoplastic left heart syndrome may also be a potential application of 3D printing.
It is important to have proper guidelines for the effective use of this technology when it is integrated into routine clinical practice. The time and risk involved in obtaining the necessary images, performing segmentation, and the cost for printing must be taken into account. Patients with simple heart defects wherein the routine imaging modalities provide a straightforward diagnosis, and appropriate treatment strategies do not require 3D printed heart models, although models of these defects may still be useful for educational purposes. These include simple atrial and ventricular septal defects, tetralogy of Fallot without associated defects, and simple transposition of the great arteries.
Image acquisition is the most important step in the process of creating a virtual model to be used to print a physical model. A significant determinant in patient selection for 3D printing is the availability of high-quality images. Currently, the imaging modalities used to derive 3D printed models include cardiac CT, CMR, and both 3D TEE and TTE. Each imaging modality has different strengths and weaknesses that impact the quality and accuracy of the 3D printed model [9]. The visualization of extracardiac anatomy and “blood pool” imaging is enhanced by CT [27]. However, nephrotoxic intravenous contrast is often required for acquisition of cardiac CT imaging datasets and exposes patients to ionizing radiation. Cumulative medical radiation is of concern and can have important health implications for young patients [28, 29]. CMR is superior to other imaging modalities for the quantification of ventricular volumes and myocardial architecture [4]. For CMR, intubation and general anesthesia are often necessary in pediatric patients. Gadolinium-based contrast may also be required for acquisition of high-resolution imaging datasets. Scanning is not possible in patients with implanted devices that are incompatible with CMR. In contrast, 3D echocardiography is a bedside tool, which is safe for severely ill patients as they do not require transportation or positioning in a scanner. Intubation and sedation are also not required except when 3D TEE is utilized or if the patient’s age makes it difficult for them to lie still for a prolonged period of time [30]. The best visualization of cardiac valve morphology is provided by 3D echocardiography when compared to other imaging modalities [31]. However, there are several limiting factors that may affect valve visualization by 3D echocardiography. Image acquisition focuses on one aspect of the anatomy, and a whole heart image dataset cannot be acquired. Technical settings including frame rate, gain, compression, and depth must be set by the echocardiographer to clearly define the blood–tissue border and to distinguish valve anatomy from artifact. Furthermore, hardware and software limitations in current ultrasound systems, specifically those affecting temporal and spatial resolutions, may not provide sufficient image quality for a 3D printed model. These limitations affect both 3D TTE and 3D TEE imaging. Availability of appropriate sized probes for TEE may be a limiting factor in young patients with complex congenital heart disease. However, 3D TEE has better image resolution and frame rates [20] and is preferable as a source dataset for 3D printing. Image acquisition is discussed in detail in subsequent chapters.
Post-processing to Virtual Model
A factor that significantly impacts the accuracy of 3D printed models is post-processing, the quality of which may vary among cardiologists and sonographers [20]. For this reason, there is a need for a unified protocol. The images from cardiac CT, CMR, and 3D ultrasound are usually acquired in the Cartesian digital imaging and communications in medicine (DICOM) format. As traditional 3D echocardiogram post-processing elements cannot be exported from segmentation software, image acquisition settings play an important role in determining the quality of ultrasound datasets. A frame rate of 30 frames per second (fps) is more than adequate for 3D echocardiography datasets. If there is no fusion artifact, 4 cardiac cycles provide optimal data for post-processing. The gain and compression settings must be optimized to get adequate visualization of the tissue–blood separation point. Visualization of the blood-tissue interface is also dependent on the patient’s size and the frequency setting of the ultrasound probe. A frequency of 5–7 MHz is usually adequate for acquisition of 3D TTE datasets for 3D printing in children.
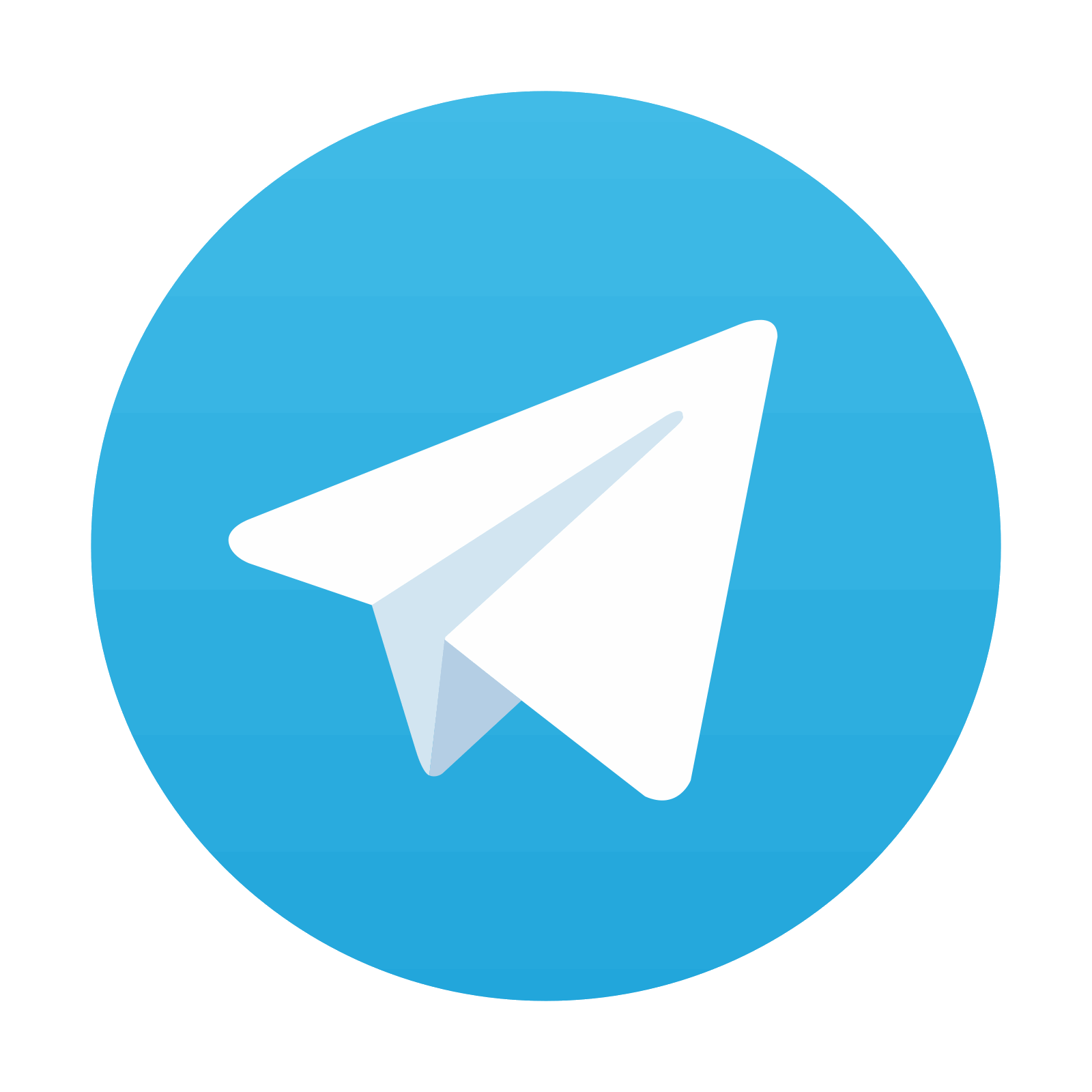
Stay updated, free articles. Join our Telegram channel
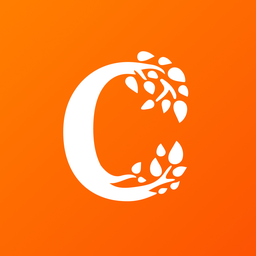
Full access? Get Clinical Tree
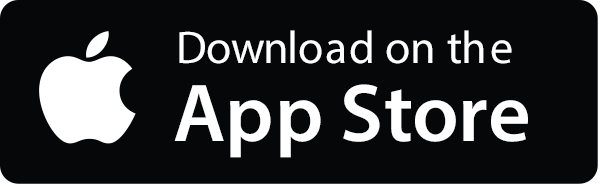
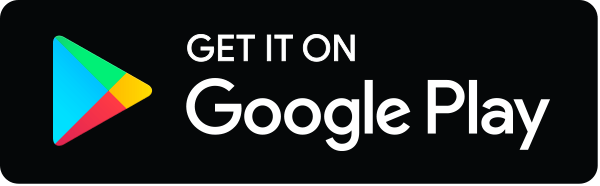