The goal of this study was to evaluate the diagnostic accuracy of transmural perfusion gradient (TPG) and transmural perfusion gradient reserve (TPGR) with 3.0 T cardiac magnetic resonance (CMR) against invasively determined fractional flow reserve (FFR) to detect coronary artery stenosis. Quantitative analysis of myocardial perfusion with CMR to diagnosis coronary artery disease (CAD) has been widely accepted. However, traditional transmural myocardial perfusion analysis with CMR neglects that endocardium is more vulnerable to ischemia than epicardium. TPG and TPGR can take the inhomogenous perfusion impairment into account and be more sensitive and specific for diagnosis of CAD. In this study, 71 patients (57 men, age 60.1 ± 6.4 years) with known or suspected CAD referred for invasive angiography study underwent rest and adenosine-induced stress CMR perfusion imaging scan. FFR was attempted to be measured in all major epicardial coronary arteries. FFR ≤0.75 was regarded to indicate a hemodynamic significant coronary lesion. A TPG ≤0.85 predicted significant CAD with sensitivity and specificity of 74.55% and 83.65%, respectively. Sensitivity and specificity of TPGR ≤0.81 were 90.91% and 89.94%, respectively. Area under the receiver-operating curve to detect FFR ≤0.75 was 0.86 for TPG and 0.95 for TPGR. TPGR yielded significantly better sensitivity and specificity for diagnosis of CAD than traditional myocardial blood flow, myocardial perfusion reserve, and TPG (p <0.0001). In conclusion, TPG and TPGR analyses with MRI are capable of detecting hemodynamic stenosis of coronary artery and superior to traditional myocardial perfusion analysis. Furthermore, TPGR appears to be superior to TPG in the diagnosis of coronary artery stenosis.
Myocardial ischemia assessment is essential for patients with suspected coronary artery disease (CAD) to guide therapeutic strategy. Most of the previous studies focused on transmural myocardium perfusion, which might overlook the early appearance of ischemia as myocardial ischemia affects the subendocardial layers earlier and more severely than the outer layers. The conventional imaging techniques such as single-photon emission computed tomography and positron emission tomography cannot provide sufficient spatial and temporal resolution. Although showing promising preliminary results, dynamic myocardial perfusion with multidetector computed tomography exposes patients to additional radiation burden. The high spatial resolution of cardiac magnetic resonance (CMR) allows the detection of subendocardial ischemia. Previous studies have revealed that transmural perfusion gradient (TPG) can be used to assess the inhomogeneous myocardial perfusion. However, diagnostic performance of transmural perfusion gradient reserve (TPGR) has not been explored yet. The objective of this study is to probe the potential diagnostic accuracy of TPG and TPGR with CMR in comparison with invasive fractional flow reserve (FFR) measurement.
Methods
Patients with suspected or known CAD referred for coronary angiography and FFR measurement were prospectively recruited and underwent CMR perfusion imaging before invasive coronary studies. Exclusion criteria included previous ST-segment elevation infarction, acute coronary syndrome <6 weeks, previous coronary artery bypass graft, impaired left ventricular (LV) function (ejection fraction <40%), estimated glomerular filtration rate <30 ml/min, contraindication to adenosine or gadolinium (Gd) contrast agents, and obstructive pulmonary disease. Patients were instructed to abstain from caffeine, methylxanthines, and smoking for 24 hours before imaging. All patients gave written informed consent in accordance with the ethical committee approval.
People were examined in a 3.0-T scanner (MAGNETOM Verio; Siemens Medical Solutions, Erlangen, Germany) using an 8-channel cardiac phased array receiver coil. The scanning protocol comprised scout film, cine functional scan, first-pass perfusion at rest and stress, and late gadolinium enhancement scar imaging. Perfusion imaging was acquired using a T1-weighted saturation recovery turbo flash sequence during injection of a bolus (equal volumes of 0.075 mmol/kg) of gadolinium-based contrast agent (gadobutrol/Gadovist; Berlin-Wedding, Schering, Germany) at 4 ml/s followed by a 20-ml saline flush by a power injector. The duration of the scan was continued for 56 cardiac cycles. Imaging consisted of 3 short-axis slices (basal, mid-papillary, and apical) covering 16 of the standard myocardial segments with the apex segment excluded. Imaging parameters: flip angle 128°, echo time 1.0 ms, acquisition window 192 ms, inversion time 100 ms, 128 phase-encoding lines, field-of-view of 340 × 265 mm 2 , and the in-plane spatial resolution 2.7 × 2.1 × 10 mm 3 . Stress imaging acquisition induced by adenosine (140 μg/kg/min for 4 minutes) preceded the rest acquisition by 15 minutes. During adenosine infusion, patient symptoms, heart rate, blood pressure, and electrocardiogram were monitored. Late gadolinium enhancement imaging was acquired 10 minutes after contrast was topped up to a total dose of 0.2 mmol gadolinium/kg of body weight.
X-ray coronary angiography was performed according to the standard methods within 2 weeks after CMR examination. FFR procedure was performed in all major epicardial coronary arteries during hyperemia induced by infusion of intravenous adenosine at 140 μg/kg/min. An FFR ≤0.75 was considered to represent hemodynamic significant coronary artery stenosis. Arteries, which were totally or subtotally occluded or had severe stenosis not appropriate for FFR measurement, were also recorded as having significant flow-limiting lesion. Coronary territories subtended by a flow-limiting coronary artery were classified as ischemic territories, and all others were classified as normal territories. Operators in the catheter laboratory were blinded to the results of the CMR scan.
Visual analysis of perfusion imaging was completed by 2 experienced observers blinded to all other data independently. The perfusion defects were defined as that stress-induced hypoperfusion were present, where visually dark areas appears because the “wash-in” dynamic of contrast enhancement was delayed, and persisted at least for 10 consecutive images, compared with a remote normal myocardial territory. If a perfusion defect occurred at stress but not at rest, it was regarded as pathological. Dark rim artifacts were recorded if rims presented both in stress and rest scans and limited to small transmural extent merely beneath subendocardium, which were excluded from further study. Late gadolinium enhancement was analyzed simultaneously to differentiate the stress-induced ischemia from scar tissue in perfusion defect areas. The hypoperfusion segments were assigned to the coronary territories according to the standard 17-segment models, excluding the apex. In case of disagreement, a third observer reviewed the images before a consensus was reached.
The implementation of high-resolution signal intensity (SI) analysis in this study preprocessed by registration to avoid respiratory and cardiac motion. The pseudo ground truth image is also calculated as template for each of the myocardial perfusion images to remove the accumulated error. Myocardial borders of LV myocardium in each short-axis imaging were outlined and manually corrected. Perfusion imaging was divided into 16 standard segments. Segments with endocardial rim artifacts, poor image quality, or late gadolinium enhancement were excluded. The mean pixel intensity was measured over time in each segment, and the region of interest was placed in the LV cavity. Spatially averaged SI values were used to plot SI versus time curves of the each segment and the region of interest. Deconvolution technique was applied to provide a parameterized myocardial blood flow (MBF) measurement using a combined programming of C++ and MATLAB developed by our group, based on the central volume principle suggested by Zierler. The exponential model was used to match the residual function as R ( t ) = P × e − t/τ , where we calculated MBF. Myocardial perfusion reserve (MPR) was calculated by dividing the value of the MBF during the stress state by the rest state.
We then subdivided each LV segments into endocardial and epicardial parts equally. The endocardial and epicardial SI versus time curves were obtained from the inner and outer halves of the LV myocardium, respectively, and corresponding MBF values (MBF endo and MBF epi ) were calculated based on previous description. The TPG was defined as the ratio of MBF endo and MBF epi . TPGR was defined as the ratio of TPG in stress and rest sate. The segments were assigned to the respective coronary perfusion territory based on the standardized LV segmentation. For each parameter we discussed, the mean value of the 2 lowest scoring segments for each coronary territory was used for further analysis and compared with FFR.
The IBM SPSS Statistics (version 21.0; SPSS, Chicago, Illinois) and Medcalc software (Med-calc, Mariakerke, Belgium) were used. Data are presented as mean ± SD, except where stated. Group means were compared using paired and unpaired Student t test as appropriate. Receiver-operating characteristic (ROC) analysis was used to assess the diagnostic accuracy of TPG and TPGR and to determine their threshold with the greatest sensitivity and specificity to detect coronary disease at an FFR ≤0.75. Optimal cutoffs were determined by the maximum Youden index. Comparisons of diagnostic accuracy of TPG analysis and tradition myocardial perfusion were performed using ROC curve analyses. ROC curves were compared using the DeLong test. Pearson correlation was used to assess correlation; p ≤0.05 was judged statistically significant in all cases.
Results
Of the 76 patients recruited to the study, 5 patients were excluded (1 had claustrophobia, 2 refused to undergo FFR measurement, and 2 with aborted FFR procedure because of vasospasm). The study protocol was completed in 71 patients. Patient demographics are provided in Table 1 . A total of 213 coronary arteries were available for analysis. Hemodynamic status changes after adenosine stress both in CMR acquisition and FFR procedure are listed in Table 2 . Stressing heart rate was significantly higher than resting (p <0.01).
Data (n=71) | |
---|---|
Age (years) | 60.1±6.4 |
Man | 57 (80%) |
Previous percutaneous coronary intervention | 6 (9%) |
Hypertension | 41 (8%) |
Diabetes mellitus | 22 (31%) |
Previous stroke | 3 (4%) |
Smoker | 43 (61%) |
Family history of coronary artery disease | 22 (31%) |
Hyperlipidemia | 44 (62%) |
Medications | |
Statin | 40 (56%) |
Beta-blocker | 32 (45%) |
Aspirin | 56 (79%) |
Clopidogrel | 5(7%) |
Nitrate | 29 (41%) |
Calcium-channel blocker | 25 (35%) |
Angiotensin-converting enzyme inhibitor or angiotensin receptor blocker | 27 (38%) |
Data (n=71) | |
---|---|
Cardiac magnetic resonance data | |
Hemodynamic status | |
Resting systolic blood pressure (mmHg) | 141±22 |
Resting diastolic blood pressure (mmHg) | 76±15 |
Resting heart rate (beats/min) | 67±10 |
Adenosine stress systolic blood pressure (mmHg) | 131±19 |
Adenosine stress diastolic blood pressure (mmHg) | 73±19 |
Adenosine stress heart rate (beats/min) | 83±13 |
Ejection fraction (%) | 54±13 |
Late gadolinium enhancement | |
Full thickness | 0 |
Partial thickness | 3 (4.2%) |
Angiographic data | |
Time from cardiac magnetic resonance scan (days) | 13.2±2.8 |
Hemodynamic status | |
Resting systolic blood pressure (mmHg) | 139±21 |
Resting diastolic blood pressure (mmHg) | 78±14 |
Resting heart rate (beats/min) | 69±11 |
Adenosine stress systolic blood pressure (mmHg) | 130±17 |
Adenosine stress diastolic blood pressure (mmHg) | 74±16 |
Adenosine stress heart rate (beats/min) | 81±15 |
Patients with coronary disease (fractional flow reserve ≤0.75) | 39 (54.9%) |
One-vessel disease | 27 (69.2%) |
Two-vessel disease | 8 (20.5%) |
Three-vessel disease | 4 (10.3%) |
Coronary arteries with fractional flow reserve ≤0.75 | 55 (25.8%) |
Left anterior descending | 20 (36.4%) |
Left circumflex | 9 (16.4%) |
Right | 26 (47.3%) |
Angiography was performed within 13.2 ± 2.8 days after the CMR, and the results are shown in Table 2 . An FFR ≤0.75 could be detected in 32 patients (45.1%). Another 2 patients had totally occluded vessels, 3 patients had subtotally occluded lesions, and 2 patients had severe calcified arteries. All those 7 patients who were unsuitable for FFR measure were also considered with hemodynamically significant lesions. Therefore, 39 patients (54.9%) were defined as having CAD.
Mean scanning time was 43 ± 14 minutes, and there were no complications from the adenosine. All perfusion studies were suitable for visual analysis. Perfusion was reported as abnormal for 56 coronary territories (26.3%) and normal for 157 territories. The FFR in vessels with a visually detected perfusion defect was 0.60 ± 0.17 versus 0.82 ± 0.17 (p <0.001) in those with visually normal perfusion. Although 42 of the 55 territories with an FFR ≤0.75 stenosis had a perfusion defect by visual CMR analysis, 14 visual perfusion defects in CMR were reported in 158 territories that were angiographically normal. Sensitivity and specificity of CMR perfusion visual analysis to detect coronary ischemia at a threshold of FFR ≤0.75 stenosis were 76.36% and 91.14%, respectively; 3 patients (4.2%) had evidence of subendocardial scar based on the late gadolinium enhancement images, and the corresponding segments were excluded from further analysis.
Twenty-six perfusion segments (2.4%) were excluded from further quantitative analysis because of endocardial dark rim artifacts in 16 segments and insufficient image quality in 10 segments. Quantitative analysis was available for all territories, and results were summarized in Table 3 . MBF and MPR of both endocardial and transmural were lower in stenotic territories compared with remote nonstenotic territories (p <0.01). However, in the analysis of epicardium, the MBF epi was not significantly different from stenotic territories and nonstenotic territories (p = 0.070). Results of ROC analysis were provided in Table 4 . The area under the receiver-operating curve (AUC) for stress MBF endo and MPR endo were significantly higher than for stress MBF trans (p <0.01) and MPR trans (p <0.001), respectively, on a vessel basis for FFR ≤0.75 ( Figure 1 ).
FFR >0.75 (n=158) | FFR ≤0.75 (n=55) | P value | |
---|---|---|---|
Stress | |||
MBF endocardial | 2.05±1.02 | 1.37±0.67 | <0.001 |
MBF epicardial | 2.04±0.90 | 1.85±0.96 | 0.070 |
MBF transmural | 2.03±0.92 | 1.59±0.79 | 0.002 |
TPG | 1.02±0.19 | 0.77±0.13 | <0.001 |
Reserve | |||
MPR endocardial | 2.24±0.95 | 1.28±0.53 | <0.001 |
MPR epicardial | 2.22±0.85 | 1.89±0.73 | 0.009 |
MPR transmural | 2.22±0.85 | 1.56±0.62 | <0.001 |
TPGR | 1.03±0.23 | 0.68±0.11 | <0.001 |
Cut-off | AUC | Sensitivity (%) | Specificity (%) | |
---|---|---|---|---|
Stress | ||||
MBF subendocardial | 1.39 | 0.77 | 74.55 (61.0-85.3) | 77.36 (70.1-83.6) |
MBF subepicardial | 1.75 | 0.64 | 52.73 (38.8-66.3) | 77.99(70.7-84.2) |
MBF transmural | 1.56 | 0.71 | 63.64 (49.6-76.2) | 79.25 (72.1-85.3) |
TPG | 0.85 | 0.86 | 74.55 (61.0-85.3) | 83.65 (77.0-89.0) |
Reserve | ||||
MPR subendocardial | 1.60 | 0.83 | 78.18 (65.0-88.2) | 75.47 (68.0-81.9) |
MPR subepicardial | 2.22 | 0.61 | 72.73 (59.0-83.9) | 50.31 (42.3-58.3) |
MPR transmural | 1.98 | 0.73 | 80.00 (67.0-89.6) | 57.86 (49.8-65.6) |
TPGR | 0.81 | 0.95 | 90.91 (80.0-97.0) | 89.94 (84.2-94.1) |
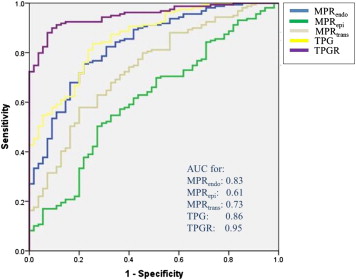
All perfusion segments satisfied early quantitative analysis were available for gradient analysis. The stress TPG and TPGR in the ischemic territories were significantly lower compared with those in normal territories (TPG: p <0.001; TPGR: p <0.001). Analysis repeated within left anterior descending artery (LAD), left circumflex artery (LCX), and right coronary artery (RCA) territories revealed similar results ( Table 5 ). Corresponding ROC analysis and scatter plot analysis for TPG and TPGR were provided in Figures 1 and 2 . ROC analysis results for LAD-, LCX-, and RCA-perfused myocardial segments remained similar ( Table 5 , Figure 3 ). In the ROC analysis, the AUC for TPG analysis to detect significant CAD was significantly higher compared with transmural myocardium perfusion analysis (TPG vs stress MBF trans : p = 0.009; TPGR vs MPR trans : p <0.001). TPGR yielded significantly better sensitivity and specificity of CAD diagnosis compared with stress TPG (p <0.0001) for predicting FFR ≤0.75 of the supplying coronary artery ( Figure 1 ). Clinical applications of gradient analysis were provided in Figure 4 .
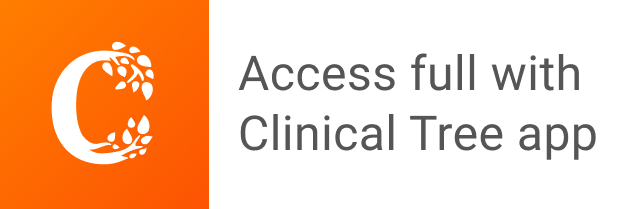