Abstract
The number of children who require chronic mechanical ventilation has grown with improvements in care, assistive technologies, and philosophical approaches to some progressive diseases. The causes of chronic respiratory failure include respiratory pump dysfunction, abnormal respiratory drive, and airway, parenchymal, or pulmonary vascular disorders. The goals of chronic mechanical ventilatory support, however, are independent of the etiology of respiratory failure. Children considered for chronic mechanical ventilation at home must be medically stable, have a family committed to learning all aspects of the child’s care, and adequate funding to support the cost of equipment, supplies, and skilled care. Technological improvements in equipment have broadened options to improve patient-ventilator synchrony and comfort. Outcomes of ventilator-assisted children depend in part on the etiology of their underlying illness, with those diseases likely to improve over time also being the most likely to permit liberation from chronic ventilatory support. Underlying disease impacts survival as well, although some children with chronic respiratory failure die of complications from other comorbidities or from tracheostomy-related accidents. Ventilator-assisted childrens’ quality of life is generally good, but their care is stressful for their families. Challenges in developing better equipment for very young patients, and in supporting patients and their families to gain better access to public resources like school, transportation, and housing as well as services to avoid caregiver fatigue, take on increased importance as this population continues to grow. Health care systems must also develop transition programs for older ventilator-dependent children as they reach adulthood.
Keywords
mechanical ventilation, noninvasive ventilation, tracheostomy, home care, positive pressure ventilation
Introduction
Children who require technology for chronic respiratory insufficiency or failure constitute a small but varied group. The US Congress’s Office of Technology Assessment (OTA) defines a technology-dependent child as “one who needs both a medical device to compensate for the loss of a vital body function and substantial and ongoing nursing care to avert death or further disability.” This definition does not take into account either site of care (hospital, home, or skilled facility) or credentials of the caregiver (professional nurse or trained layperson). Further, the OTA identifies four separate groups of children that would be considered technology-dependent. Two of these include children dependent on mechanical ventilation for at least part of the day and children dependent on other device-based respiratory support such as tracheostomy tubes, airway suctioning, and the use of supplemental oxygen. This chapter discusses only those children requiring technology for the treatment of chronic respiratory failure, a condition for which mechanical ventilatory support is required for at least 4 hours/day for a month or longer.
Epidemiology
The large-scale need for chronic respiratory support of children began with the polio epidemics of the 1930s, 1940s, and 1950s. The development of subspecialties in neonatology and pediatric critical care in the 1960s resulted in advances in medical therapies that allowed children with previously fatal conditions to survive, albeit occasionally with chronic conditions such as respiratory failure. A focus on pediatric chronic respiratory failure did not occur in the United States until the early 1980s. In 1981, President Ronald Reagan waived the eligibility rules for Supplemental Security Income to allow Katie Beckett, a ventilator-dependent child, to be cared for at home without losing Medicaid benefits, thus bringing the population of children with chronic respiratory failure into the national spotlight. In 1982, the surgeon general then convened the Workshop on Children with Handicaps and Their Families, where he noted that advances in medical interventions created a new type of disability: infants chronically dependent on ventilatory support as a result of other medical interventions, which he referred to as “a creature of our new technology.”
The causes of chronic dependency on mechanical ventilation or supplemental oxygen can be categorized as (1) those that affect the respiratory pump (respiratory muscles, rib cage, ventral abdominal wall); (2) those that affect respiratory drive; (3) extrathoracic and central airway lesions; and (4) pulmonary parenchymal and vascular abnormalities ( Table 21.1 ). Individual patients can have more than one cause for chronic ventilator dependency (i.e., neuromuscular weakness and severe kyphoscoliosis, or anoxic encephalopathy with abnormal respiratory drive, recurrent aspiration, and obstructive sleep apnea).
Respiratory pump |
|
Respiratory drive |
|
Airway |
|
Pulmonary parenchymal and vascular problems |
|
In the United States, there is no national registry that tracks the population of ventilator-assisted individuals. Center-specific surveys of children cared for in acute pediatric hospitals in the 1980s demonstrated that bronchopulmonary dysplasia (BPD) and noncardiac congenital anomalies were the most common diagnoses of infants and toddlers who required prolonged mechanical ventilation, whereas spinal cord injury and underlying neuromuscular diseases were more common among those cared for in rehabilitation facilities. In Massachusetts, surveys of children receiving chronic mechanical ventilatory support outside of acute care hospitals showed that the chief cause of chronic respiratory failure (CRF) was sequelae of prematurity or congenital malformations in the 1980s and early 1990s. However, over the next 15 years the major causes of CRF became neuromuscular disease and central nervous system injury or malformations. In addition, the number of children receiving such care outside a hospital had nearly tripled from 1990 to 2005. National surveys from Canada, Europe, and Japan have similarly demonstrated that neuromuscular disease and central nervous system injuries are the most common indications for chronic ventilation in children. This probably reflects a philosophical change toward providing chronic respiratory support to patients with progressive neuromuscular disorders, an increasing role of noninvasive ventilation for these patients, and a reflection of the types of patients most commonly requiring prolonged care in pediatric intensive care units.
The prevalence of children requiring chronic mechanical ventilation has grown over the last three decades. In 1987, the OTA estimated that there were between 600 and 2000 ventilator-dependent children in the United States. Ten years later, extrapolations of a survey from Minnesota estimated that there were 17,824 ventilator-assisted individuals in the United States, of whom 5534 would be below 21 years of age. It was also shown that subjects below 20 years of age represented about one-third of all ventilator-assisted individuals in the state, with those below age 11 years being the largest group and those between 11 and 20 years accounting for the greatest increase since the prior survey of 1992. This survey included only patients in whom a backup ventilator rate was used; thus the number was likely an underestimate. In the 1990s, the prevalence of children requiring chronic mechanical ventilation was estimated to be 0.5 per 100,000 in the United Kingdom, 0.68 per 100,000 in Japan and 1.2–1.5 per 100,000 in the United States. Extrapolating data from Massachusetts and Pennsylvania, King estimated the prevalence of ventilator-assisted children cared for at home in the United States in 2010 to be 4.7–6.4 per 100,000. Recent international prevalence rates of home mechanical ventilation in children below 18 years of age are similar, ranging from 4.2 to 6.7 per 100,000. The method of mechanical ventilation has changed over time as well, with most recent surveys demonstrating a marked rise in the percentage of children supported by noninvasive ventilation and far fewer receiving ventilation via tracheostomy. The overall increase in prevalence of ventilator-dependent children cared for at home is likely multifactorial, reflecting advances in the medical care of critically ill children, improved technology in home equipment and pediatric noninvasive interfaces, increased use of polysomnography in children to detect nocturnal hypoventilation and philosophical changes in the willingness of physicians to apply these technologies to children with progressive diseases.
Goals of Therapy
The objectives of technology dependence support are largely independent of the cause of respiratory failure. They include reversing or ameliorating the cause of respiratory compromise, extending life, improving physiologic function, and reducing morbidity. In infants and young children, respiratory failure can also be associated with slow growth and developmental delay; therefore chronic ventilatory support is sometimes used to avoid these consequences. Occasionally, ventilatory support is augmented during periods of physical activity to promote the child’s potential for rehabilitation, or it may be increased if the child fails to maintain adequate growth velocity. Thus in formulating the daily prescription for mechanical ventilation, the desire to reduce support must be balanced with a more global view of the child’s needs.
Psychosocial development and quality of life can be enhanced when chronic ventilatory support is safely accomplished outside the intensive care unit. The home setting has long been considered the best place for technology dependent children in terms of their psychological and somatic development, but excellent medical, psychosocial, and developmental support, including weaning from mechanical ventilation when appropriate, can be safely accomplished in inpatient rehabilitation facilities (within or outside the acute care hospital). The setting for postacute care will depend on the degree of medical stability of the patient, the willingness and ability of family caregivers to provide care in the home, and community resources. Although the number of ventilator-dependent children has increased over the years and home care of this population has become more common, it should never be assumed de facto that home care is the best setting for an individual child or family. This can be concluded only once a full assessment of the child, family, resources, home care setting, and available alternative settings has been made. Additionally, the best location might change throughout a child’s course—for instance, as the child’s medical condition improves or worsens, or if caregiver availability changes.
Pathophysiology of Respiratory Failure
Respiratory failure can result from an imbalance between the output of the respiratory pump and the load against which it has to work, development of respiratory muscle fatigue, inadequate respiratory drive, pulmonary parenchymal disease or pulmonary vascular disease. The disparity between the abilities of the respiratory pump and its load refer to either inadequate pump output for the imposed load, as in the case of a child with respiratory muscle weakness, or an increased load for the output. This could be the result of a reduction in lung compliance, an increase in pulmonary resistance, or stiffening of the chest wall itself. In theory, “pump failure” results in hypercapnia, whereas parenchymal disease causes hypoxemia. In practice, however, the two often coexist, and hypoventilation from pump failure will result in hypoxemia. An understanding of the causes of respiratory failure often provides a rationale for the type of assisted ventilation that will provide the best support for the individual. It is critical to recognize that although chronic mechanical ventilation will not alter the ultimate prognosis for chronic progressive disorders like Duchenne muscular dystrophy, spinal muscular atrophy (SMA), or cystic fibrosis, its use can still prolong survival, relieve dyspnea, and improve daytime function and quality of life for children with such disorders.
Inadequate Pump Output (e.g., Neuromuscular Disease, Spinal Cord Injury)
There is a typical progression of respiratory morbidity in patients with neuromuscular disease (NMD). Once weakness of respiratory muscles is present, airway clearance becomes compromised, predisposing patients to recurrent episodes of lower respiratory tract infections and atelectasis. This results in a decrease in lung compliance and therefore an increase in the load against which the respiratory pump must work. Infants and young children with NMD are more likely to experience complications from mucus plugging because their airways are smaller and peripheral airways contribute a greater proportion of intrathoracic resistance in children under 5 years. Early in childhood, the chest wall of children with NMD is abnormally compliant ; as a result, the effect of chest wall recoil on lung volume and airway caliber is reduced, and this contributes to intrathoracic airway narrowing.
There are also ventilatory consequences of this alteration of chest wall compliance. In the young child with neuromuscular weakness and a highly compliant chest wall, much of the work of the respiratory muscles can be wasted as chest wall retractions reduce the contribution of the thoracic compartment to ventilation. Contraction of intercostal muscles can help to maintain tidal volume, but at the cost of increased respiratory energy expenditure; thus adequate ventilation can be maintained, but growth failure might ensue. In very young children, the imbalance between the highly compliant chest wall and relatively stiff lungs can result in an acquired pectus excavatum deformity. Acquired chest wall deformity like this has led to the theoretical concern that when chronic low-volume breathing is present in infants, it can also impair lung growth and development, although this has never been demonstrated. Nevertheless, noninvasive ventilation has been used to correct or prevent the development of pectus excavatum in young children with NMD.
Over time, the chest wall of subjects with neuromuscular weakness becomes stiffer than normal because of prolonged periods of low-tidal-volume breathing without deep sigh breaths. This leads to the development of ankylosis around costovertebral joints, with stiffening of ligaments and tendons. When present, the progression of kyphoscoliosis will contribute to a mechanical disadvantage around the costovertebral joint, making the respiratory muscles less efficient. The sum of these imbalances results in a restrictive pattern of lung function, with reduced vital capacity and total lung capacity. If subjects have significant expiratory muscle weakness, however, the residual volume and functional residual capacity can be normal or even slightly elevated, because the rib cage cannot be reduced below its normal resting volume.
As weakness progresses, patients experience episodes of hypoventilation during sleep, especially during rapid-eye-movement (REM) sleep. Obstructive apnea can also occur because of weakness of the bulbar musculature or physical features of certain neuromuscular diseases such as macroglossia, retrognathia or flattening of the malar eminences. When respiratory muscle weakness becomes sufficiently severe to cause hypoventilation during sleep, the patient will compensate with an arousal response. This protective mechanism causes sleep disruption and fragmentation, poor sleep quality, and reduced sleep time. These phenomena, in turn, can cause daytime somnolence and fatigue as well as poor school performance. Initially, patients will demonstrate hypercapnia during sleep, but they will be able to maintain normocapnia while awake. As the severity of sleep-disordered breathing increases, either because of prolonged periods of nocturnal hypoventilation, mechanical alterations of the respiratory system or both, central drive becomes blunted and the patient does not respond in an appropriate way to the challenge of elevated PaCO 2 . At this point, diurnal hypercapnia will occur, but the daytime hypercapnia can be corrected if nocturnal mechanical support of breathing is instituted. Further progression of weakness, however, will result in ineffective ventilation during the day and diurnal hypercapnia, even when nocturnal ventilation has been instituted.
Timing of the progression of respiratory failure varies with different NMDs. In the absence of ventilatory support, infants with SMA who are unable to sit typically die by 2 years of age. Boys with Duchenne muscular dystrophy, in contrast, do not exhibit respiratory compromise until their second decade of life. Respiratory muscle weakness in congenital myopathies is often static, but as children grow, their status may deteriorate because the respiratory muscles cannot compensate for the increase in body mass. Respiratory failure often ensues during the pubertal growth spurt. Some disorders, like myotonic dystrophy or myelomeningocele with Chiari malformation, are associated with primary disorders of ventilatory control.
Paralysis of the diaphragm results from cervical injury above C-3 or from phrenic nerve injury, often in the setting of birth trauma, cardiac surgery or other thoracic surgery. Infants can occasionally compensate for unilateral paralysis, but bilateral diaphragmatic paralysis requires ventilatory support. When unilateral diaphragmatic paralysis causes respiratory failure, plication of the affected diaphragm often improves ventilation sufficiently that mechanical support is not required. If the paralysis is the result of trauma, plication does not prohibit recovery of function of the diaphragm.
Abnormal Respiratory Pump (e.g., Early-Onset Scoliosis, Asphyxiating Thoracic Dystrophy, Severe Kyphosis)
The respiratory pump itself can present a load too great to be overcome by the muscles of respiration. Distortion of the thoracic cage from early-onset scoliosis results in a restrictive pattern of lung function. A reduction in chest wall compliance can occur when ribs are congenitally fused, the intercostal muscles are replaced by fibroconnective tissue after years of disuse or when costovertebral joints become ankylosed from chronic low-tidal-volume breathing without sigh breaths. Alternatively, distortion of the thoracic cage can place the inspiratory muscles at sufficient mechanical disadvantage that their force-generating ability is compromised. Then the thoracic compartment is noncompliant, and the majority of the volume change that occurs with ventilation is in the abdominal compartment. Thoracic insufficiency syndrome is a term used to describe conditions where the thorax cannot support normal ventilation or lung growth. Since the volume of the thorax is small in these patients, tidal volume targets during mechanical ventilation are smaller as well.
Abnormal Respiratory DrivE (e.g., Congenital Central Hypoventilation Syndrome, Chiari Malformation, Brain Stem Lesions, Leigh Disease)
Several rare disorders result in an abnormal response to hypercapnia and hypoxemia. Congenital central hypoventilation syndrome (CCHS) represents a failure of autonomic control of breathing; it is caused by a defect in the paired homeobox 2B (PHOX2B) gene, which produces a transcription factor essential to migration of neural crest cells and development of the autonomic nervous system. There is a range of ventilatory abnormalities in children with CCHS, so that some children present in the neonatal period with chronic alveolar hypoventilation, requiring around-the-clock ventilatory support, while others may not present until adulthood. Often, as infants grow older, they are able to breathe adequately without support while awake but require ventilatory assistance during sleep and with acute illnesses. Because patients with CCHS do not respond appropriately to hypoxemia or hypercarbia, their mechanical ventilation strategy requires a mandatory rate to assure adequate ventilation. Since the underlying lung parenchyma and chest wall are normal, the delivered tidal volume should be normal as well.
Prader Willi syndrome and rapid onset obesity with hypothalamic dysfunction, hypoventilation, and autonomic dysregulation (ROHHAD) represent two other disorders associated with potentially severe hypoventilation. The majority of children with Prader-Willi syndrome have a deletion of the long arm of chromosome 15, whereas the genetic abnormality leading to ROHHAD is currently unknown. Both conditions are associated with hyperphagia and the rapid onset of obesity, but children with ROHHAD also have problems with salt and water balance. The presence of obesity in both conditions can also cause obstructive sleep apnea and contribute to sleep-related hypoventilation. Obesity hypoventilation syndrome—defined as a body mass index (BMI) greater than 30 kg/m 2 , daytime hypercapnia and hypoxemia, and sleep-disordered breathing—is another cause of respiratory failure in children, although its pathophysiology is not well understood.
Children with Chiari malformation demonstrate a blunted response to hypercapnia, suggesting a central chemoreceptor problem. They may also have both central and obstructive apnea during sleep. Thus assuring adequate minute ventilation and providing necessary distending pressure to overcome upper airway obstruction are both important strategies in supporting these patients. If the Chiari malformation is accompanied by myelomeningocele and kyphoscoliosis, adjustment of the tidal volume will be necessary.
Parenchymal Lung or Intrathoracic Airway Disease (Bronchopulmonary Dysplasia, Cystic Fibrosis, Tracheomalacia, Bronchiectasis)
The majority of children in this category of respiratory failure are those with chronic sequelae of neonatal lung disease. Approximately 1.5% of all newborns develop bronchopulmonary dysplasia (BPD) (chronic lung disease of infancy), making it the most common cause of chronic obstructive lung disease among infants. A recent point prevalence study showed that 36.5% of infants hospitalized in eight tertiary referral neonatal intensive care units fulfilled criteria for severe BPD, defined as those born after less than 32 weeks’ gestation and receiving ≥30% or more supplemental oxygen and/or positive pressure ventilation at 36 weeks postmenstrual age (PMA). Among the infants with severe BPD, 41% were still supported by positive pressure ventilation at 36 weeks PMA, suggesting that they are at greater risk to require prolonged mechanical ventilation. BPD is associated with lung hypoplasia resulting from the arrest of alveolar development, but there is also evidence of parenchymal scarring, air trapping and distortion of lung architecture. The central airways can become deformed from cyclic positive pressure distension, leading to acquired tracheomalacia or bronchomalacia. These abnormalities lead to a decrease in lung compliance and conductance, increasing the respiratory pump load. Severe air trapping can impair diaphragm contractility by altering its length-tension relationships. These can all result in an unfavorable balance between respiratory pump output and load, leading to chronic respiratory failure and a need for prolonged mechanical ventilation. The energetics of breathing may comprise as much as 25% of the infant’s caloric needs. Thus, if weaning from mechanical ventilation is too aggressive, some infants with BPD can maintain adequate gas exchange, but only at the expense of growth and development. The need for supplemental oxygen will depend on the extent of parenchymal damage, hypoplasia, or ventilation/perfusion mismatch. Most infants with BPD who require chronic mechanical ventilation develop respiratory failure while in the neonatal intensive care unit and do not recover before the time when they are otherwise considered ready for discharge. Occasionally, however, chronic respiratory failure ensues after discharge in the setting of an acute viral lower respiratory illness. Prolonged mechanical ventilation is appropriate in both settings, as lung function is expected to improve with growth and postnatal lung development.
In recent years chronic mechanical ventilation of patients with cystic fibrosis (CF) has been performed, first as a bridge to lung transplant and subsequently as a palliative measure. Like other patients whose respiratory pump becomes inadequate for the load, patients with CF and severe airway obstruction develop a rapid, shallow breathing pattern that is inefficient. When challenged with an increased load or imposed hypercapnia, the mechanics of the respiratory system limit the patient’s response. As obstruction worsens, dynamic compliance of the lung falls as well, contributing to an increased respiratory load. At the same time, downward displacement of the diaphragm from hyperinflation places it in a less favorable mechanical position, contributing to inefficiency of the respiratory pump.
General Considerations for Home Care
Often, the need for chronic mechanical ventilatory support is determined following an acute illness, when the child is unable to wean from mechanical ventilation. In other children, the requirement for chronic mechanical ventilation is recognized following an abnormal polysomnogram or identification of other nonacute indicators such as poor growth or chronic dyspnea. No matter the presentation, once it has been decided that chronic mechanical ventilation is necessary for the support of a child, the eventual site of long-term care must be determined. This requires assessment not only of the medical stability and suitability of the child but also of the family as potential medical caregivers. Other decisions regarding the approach to the child’s care (e.g., the performance of a tracheostomy) require careful consideration as to how the choice of the child’s site of care will be affected.
A child’s medical stability must be evaluated within the context of the site of care. The required level of stability at the time of transfer from a pediatric intensive care unit (PICU) will differ between home and a care site within a hospital or a subacute care facility ( Table 21.2 ). In any setting, the child must have a stable airway (natural or tracheostomy), have blood gas values that are appropriate for the underlying diagnosis, and not have care demands that exceed the resources of the site of care. When a child goes home supported by mechanical ventilation for the first time, there should be no changes in the medical plan for at least 1 week before discharge to assure that the child is adequately supported on the proposed regimen. In addition, any change to the medical regimen within 1 week of the initial hospital discharge has been associated with unanticipated readmission within 1 month of the child’s going home.
Home | General Hospital Ward/Transitional Care Facility |
---|---|
CLINICAL | |
Positive trend on growth curve | No need for 1 : 1 nursing care |
Stamina for periods of play | No invasive monitoring |
No frequent fevers or infections | No need for intravenous vasopressors or vasodilators |
PHYSIOLOGIC | |
Stable airway | Mature tracheostomy ≥ 1 week postoperatively |
PaO 2 ≥60 torr in FiO 2 ≤0.4 | SpO 2 >92% in FiO 2 ≤0.40 |
PaCO 2 <50 torr (parenchymal disease) or <45 torr (chest wall or neuromuscular disease) | Stable blood gases within normal range for the diagnosis |
No need for frequent ventilator setting changes | Stable ventilator settings ≥1 week |
When a technology-dependent child is being discharged to home, two adults must be willing and able to learn and assume all aspects of the child’s daily care, including dosages and indications for all medications being used, feedings, respiratory assessment, ventilator assessment and troubleshooting, and equipment care. For children with weak or ineffective cough, caregivers must learn various techniques of airway clearance to avoid atelectasis or treat acute illnesses. If the child will receive mechanical ventilation through a tracheostomy, the family caregivers must also learn how to suction the artificial airway and perform routine and emergency tracheostomy tube changes. In addition, there must be adequate financial support from third-party payers to provide the equipment and supplies necessary to care for the child at home. The residence in which the child will be cared for must have adequate space for the child, equipment, and visiting health care providers. The home must have running water, heat, electricity and a working telephone. Entrances must be accessible for a patient confined to a wheelchair.
The amount of skilled nursing care the family will require must also be included in the discharge plan. All families of children who cannot correct an airway or ventilator problem, or call for help, should be offered skilled nursing care for at least a portion of the day to allow caregivers to sleep with the reassurance that the child’s welfare is not at risk. Funding for those services, which are the most expensive component of the home care of technology-dependent children, should be guaranteed by third-party payers with periodic reassessments established to determine ongoing needs. In some health care systems outside of the United States, personal care attendants rather than nurses are funded to perform some of these services and to facilitate the patient’s full participation in society at a lower cost. Although there are no uniform criteria for establishing the number of nursing hours provided, this should be determined by the medical needs of the child, the capabilities of the family, and other demands on family providers (work, other children in the home, etc.). Funded respite care, to allow caregivers time off from continuous medical care and monitoring of the child, should also be built into the discharge plan, as this has repeatedly been identified as an essential component to help relieve stress and caregiver burnout.
Treatment of Chronic Respiratory Failure
Noninvasive Ventilation
Noninvasive ventilation (NIV) refers to correction of alveolar hypoventilation or upper airway obstruction without the use of artificial airways (endotracheal or tracheostomy tubes). It is usually used at night, rather than continuously, but if a child has a progressive disorder or experiences an acute decompensation, NIV can be used 24 hours/day. For patients with NMD or chest wall restriction who develop symptomatic hypercapnic respiratory failure, 4 hours or more of nocturnal NIV per day can cause a sustained decrease in daytime PaCO 2 and resolution of symptoms related to sleep-disordered breathing. For instance, nocturnal NIV corrected diurnal hypercapnia and severe nocturnal hypoventilation and maintained normal daytime PaCO 2 for an extended period in a group of 23 young men with Duchenne muscular dystrophy. In this series, nocturnal NIV was associated not only with improved survival (85% at 1 year, and 73% from 2 through 5 years) but also with sustained improvement in daytime gas exchange throughout the 5-year duration of the study.
The proposed mechanisms by which nocturnal NIV improves overall function in patients with restrictive disorders include resting of the respiratory muscles to reverse fatigue, improving lung and chest wall mechanics by reversing areas of microatelectasis, increasing chest wall excursion and resetting central chemoreceptor sensitivity to CO 2 . These mechanisms are not necessarily mutually exclusive, but evidence that NIV improves lung or chest wall mechanics in a sustained way is lacking. Several series involving subjects with a variety of neuromuscular diseases failed to demonstrate an increase in vital capacity after the institution of NIV, and the institution of NIV in young men with Duchenne muscular dystrophy before the onset of respiratory failure failed to slow the expected decline in vital capacity. It may be that episodic chest inflations closer to total lung capacity, or “lung volume recruitment maneuvers,” are required to alter the decline in vital capacity. Furthermore, direct measurements of lung and chest wall compliance after 3 months of NIV did not change in a series of 20 patients with neuromuscular disease and restrictive defects. By contrast, the investigators demonstrated enhanced responsiveness to CO 2 in these subjects after 3 months of NIV, suggesting a resetting of central chemoreceptors and enhancement of respiratory drive. Another study showed that nocturnal NIV can restore CO 2 responsiveness by an alternate method: reduction of respiratory muscle fatigue and improvement in respiratory muscle endurance. Investigators calculated the tension time index of the respiratory muscles, a noninvasive measurement of the likelihood of muscle fatigue, in 50 subjects with Duchenne muscular dystrophy just after they ended a night of NIV support (8 a.m.) and again just before they resumed nocturnal NIV (8 p.m.). To assess respiratory muscle endurance, the authors also measured the time to fatigue ( T lim ) after pressure threshold loading under the same conditions. There was a progressive increase in the tension time index and a decrease in T lim that correlated with the progression of symptoms. In the most affected subjects, however, the tension time index decreased and T lim increased significantly following a night of NIV support. Thus these data suggest that nocturnal NIV can unload weakened respiratory muscles, thereby improving their daytime capacity and reducing their fatigability.
Although noninvasive ventilation is usually confined to those who require support for 16 hours/day or less, it has been used successfully in patients who require continuous support. This can be achieved either by a nasal interface or by mouthpiece. Contraindications include poor glottic function, inability to achieve adequate ventilation noninvasively, patient preference, and lack of caregiver expertise. However, sometimes NIV has been used successfully in infants with SMA and children with static encephalopathy who have impaired glottic function. The use of NIV has been recommended by some over ventilation via tracheostomy for palliation of dyspnea in severely affected infants with SMA type I.
Complications of positive-pressure NIV are related to either the interface itself or misdirection of the applied pressure. Choices of nasal or oronasal interfaces for infants and small children are limited, so adaptation of adult interfaces is often necessary. Poorly fitting interfaces lead to leaks that not only interfere with adequacy of ventilation but also can cause eye irritation. In response, straps are often tightened to reduce the leak. This can cause facial erythema and skin ulceration. Use of a custom-molded mask or an alternate interface with different pressure points can reduce this complication, but attention to skin integrity is an integral component of care of the child receiving positive-pressure NIV. Skin ulceration can preclude the ability to provide effective NIV and force a decision to advance to tracheostomy. Prolonged application of pressure by nasal interfaces on the growing face has been associated with midface flattening. Although special devices have been created to correct this, use of more than one interface with different pressure points or a custom-molded mask should be considered.
High flows related to NIV can cause nasal congestion and mouth dryness ; these deleterious side effects can be ameliorated by humidifying the circuit. The positive pressure applied during NIV has been associated with ear and sinus pain in adults and retrograde lacrimal duct flow in children, and higher positive pressures can cause aerophagia. Gastric distension, in turn, can impair the effectiveness of NIV by creating abdominal competition for lung expansion.
Ventilation via Tracheostomy
Invasive ventilation via tracheostomy is typically used in infants and children with parenchymal lung or congenital heart disease. In addition it is used in young children who require continuous mechanical ventilation, those with severe craniofacial malformations (or other causes of upper airway or central airway obstruction that cannot be corrected by NIV) and those with severe developmental delay. Whenever possible, relatively small tracheostomy tubes are used to allow for a leak : this facilitates speech and avoids damage to the tracheal wall. When leak around the tracheostomy tube is large, however, effective mechanical ventilation can be compromised. This is especially true if the child is being ventilated in a volume-control mode, since the large leak will prevent adequate development of intrathoracic pressure to expand the chest because the ventilator breath escapes through the mouth and nose. The leak may be variable, so that even when mechanical ventilation is adequate during awake hours, significant hypoventilation can occur during sleep. This is remedied either by changing to a pressure-control mode of ventilation or by using a cuffed tracheostomy tube.
The presence of a tracheostomy increases the complexity of care for most patients requiring ventilatory assistance. Caregivers must be taught how to suction, clean and change the tracheostomy tube and how to assess for displacement and obstruction. The presence of a tracheostomy tube interferes with the child’s speech and swallowing, increases risk for infection and aspiration and is associated with airway complications such as infection at the stoma site, granuloma formation, tracheal stenosis and traumatic tracheoinnominate or tracheoesophageal fistula formation. Although the presence of a tracheostomy alone can increase caregiver stress, in some situations (such as the need for continuous ventilatory assistance and difficulty with secretion management in a young child) it can ease the burden of care. Thus the decision to advance from noninvasive to invasive ventilation must be individualized, considering the impact on both the child and the child’s caregivers.
Options for Ventilatory Support
Body Ventilators
Initially, negative-pressure body ventilators were used to augment the ventilatory efforts of patients with restrictive lung disease. Devices like the iron lung (tank ventilator), chest shell, poncho and cuirass ventilators can all successfully reverse respiratory failure in children. Negative-pressure body ventilators, however, are cumbersome and can cause upper airway obstruction. This may result from phasic collapse of the epiglottis or a lack of preinspiratory upper airway muscle activation before the ventilator supplies a negative-pressure breath. Negative-pressure ventilators are difficult to get into and out of; thus they are not well suited for subjects who require frequent access for care. They are not portable, so the user cannot readily travel or sleep at other people’s homes. Nevertheless, a negative-pressure ventilator can be an excellent alternative for the patient who cannot tolerate placement of a nasal device or the sensation of nasal positive pressure. Positive-pressure body ventilators, like the pneumobelt, are used to only a limited degree in children. The pneumobelt must be used while the patient is in a seated position so it is not suitable for the treatment of nocturnal hypoventilation.
Positive-Pressure Devices
The most common way for children to receive ventilatory assistance noninvasively is by positive pressure delivered via nasal, oronasal or mouthpiece interfaces. Application of positive pressure can relieve upper airway obstruction as well as improve minute ventilation and unload inspiratory muscles. The source of positive pressure can be a portable volume- or pressure-preset ventilator, bilevel positive-airway-pressure (B i PAP) device, or continuous positive-airway pressure (CPAP) device. B i PAP and CPAP devices use a blower to generate flow adequate to achieve the desired pressure set by the practitioner. Portable ventilators use pistons or turbines to generate the selected volume or pressure and can do so at lower flow rates. Newer positive-pressure ventilators can also provide continuous flow, which allows for spontaneous breathing without imposing additional work and dead space. In general, B i PAP units are smaller than portable ventilators, but newer portable ventilators generally weigh less than 15 pounds. B i PAP devices operate more quietly, compensate for leaks better and are also less costly than portable ventilators. On the other hand, B i PAP devices cannot generate such high peak pressures and have higher rates of energy consumption than portable ventilators. Unlike portable ventilators, most B i PAP devices do not contain an internal battery. Because they use a single-limb circuit for inspiration and exhalation, they are also more likely to promote rebreathing than systems with a double-limb circuit.
Newer portable ventilators blur some of these distinctions and combine features of standard ventilators with those of B i PAP devices. They can compensate for leaks well and are suitable for noninvasive use. They can also be adapted from “open system” single-limb circuits with passive exhalation valves and leak compensation to “closed-circuit” systems with a mechanical exhalation valve. These machines have an internal battery, the ability to control several ventilation variables and more extensive monitoring capabilities than B i PAP devices.
Some consider that CPAP is not a form of noninvasive ventilation, since the patient receives neither mandatory breaths nor assistance during spontaneous efforts. CPAP can, however, unload respiratory muscles and enhance minute ventilation by relieving upper airway obstruction, offsetting intrinsic PEEP or improving lung compliance. B i PAP devices provide pressure-support ventilation, in which the patient’s spontaneous effort is supported to a preset pressure. The supported breath is initiated by the patient, and the support is cycled off when inspiratory flow falls to a preset percent of peak flow. Many B i PAP devices have, in addition to a spontaneous mode, timed or combined spontaneous/timed modes in which mandatory breaths can be delivered in the event that the patient’s drive or ability to trigger the machine is inadequate. The sensitivity of trigger and cycle variables differs according to the manufacturer, making some machines a poor choice to use in infants or patients who are very weak. In such circumstances, the practitioner can set a mandatory rate higher than the child’s spontaneous rate to overcome the mechanical shortcomings of the system. When supplemental oxygen is required, the amount bled into the system can mask the patient’s inspiratory efforts by contributing additional flow that must be overcome to trigger the device. The final FiO 2 delivered to the patient will vary depending not only on the flow rate of oxygen bled into the system but also on where in the circuit the oxygen is introduced and the inspiratory pressure measured. It is unusual to be able to deliver an FiO 2 above 0.5–0.7 noninvasively.
Older first-generation portable ventilators, which are still in use, are able to provide only volume-preset breaths in which a desired tidal volume is set by the practitioner. The breaths can be delivered in a synchronized intermittent mandatory ventilation (SIMV) mode, where the patient breathes spontaneously but unsupported through the ventilator circuit in between mandatory positive pressure breaths delivered according to a set rate, or in Assist/Control (A/C) mode. Here the practitioner sets a rate at which mandatory breaths are delivered, but if the patient wishes to breathe above that rate, each effort results in a fully supported positive-pressure breath. Such machines also require the use of an external PEEP valve if end-distending pressure is required. When large leaks are present in children with tracheostomies who use this type of ventilator, it is usually not possible to maintain the set PEEP.
Newer second- and third-generation portable ventilators can provide either pressure preset or volume preset breaths, pressure support breaths, and continuous flow. Thus they are more versatile, allowing for more modes of ventilatory support that can enhance ventilator-patient synchrony and hence patient comfort. The PEEP valve is integrated into the machine. These machines can be used to provide CPAP with or without pressure support, SIMV with or without pressure support and A/C ventilation. Both trigger and cycle variables can be adjusted on most models. All have internal batteries that differ widely among models in duration, whereas external lightweight lithium batteries allow for their use away from an electrical power source for several hours.
No single type of positive-pressure device is ideal for all patients. Patient characteristics, as well as machine characteristics like trigger and cycle sensitivities, and sensitivity to leaks, can influence the degree of patient-ventilator dyssynchrony and adequacy of ventilatory assistance. Patients who breathe spontaneously will benefit from features like continuous flow and pressure support. Patients with no spontaneous respiratory effort (e.g., children with cervical spinal cord injury) do not require such accessories, and their presence can occasionally be detrimental. When there is a large leak around the child’s tracheostomy or interface, the leak will be interpreted by the ventilator as a patient-initiated breath and will result in unintended excessive ventilation or ventilator auto triggering. If trigger or cycle sensitivities are not adequate, the child will make inspiratory efforts that are not supported, or have to exhale while the device continues to provide a positive pressure breath. In the best of circumstances the needs of the patient are matched to the capabilities of the device, and interactions between device and child are assessed critically before the child is given that machine for long-term use.
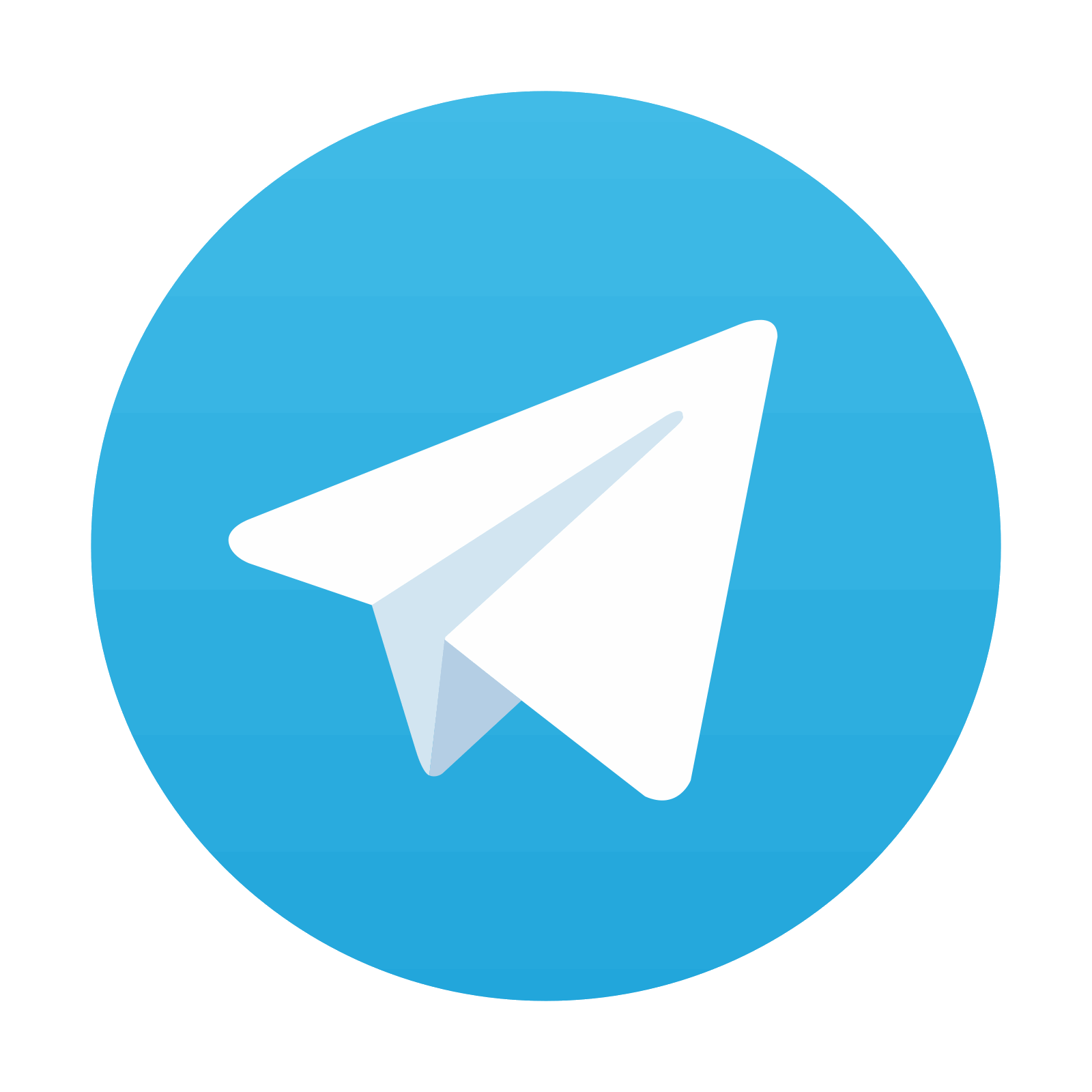
Stay updated, free articles. Join our Telegram channel
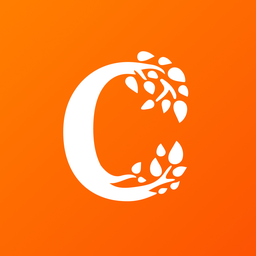
Full access? Get Clinical Tree
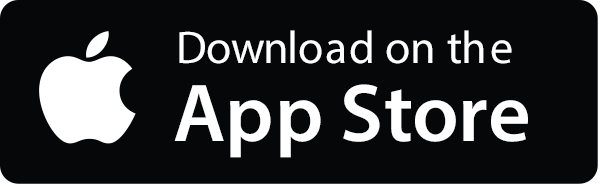
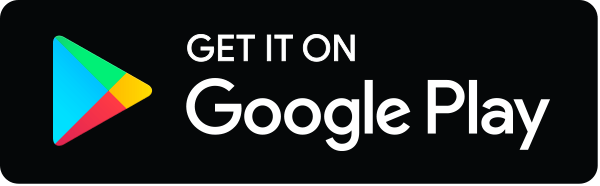