Abstract
Disorders of the chest wall, spine, and respiratory muscles include an array of conditions which share respiratory function abnormalities consistent with restrictive lung disease. Depending on the cause, they can manifest from birth to adolescence, with a very variable prognosis ranging from death during infancy (e.g., with severe congenital chest wall deformity or spinal muscular atrophy) to milder functional abnormality later in life (e.g., adolescent kyphoscoliosis). Neuromuscular diseases require annual assessment of respiratory function. In addition, polysomnography or combined pulse oximetry and transcutaneous/end tidal PCO 2 is necessary, especially when vital capacity is lower than 60% predicted value. Lung inflation techniques and mechanical cough assist are the mainstay of treatment for neuromuscular diseases, as well as noninvasive mechanical ventilation, which can now be delivered at night or around-the-clock. These “respiratory aids” have revolutionized the care and prognosis of such patients, as well as their quality of life.
Keywords
chest wall, respiratory muscles, neuromuscular diseases, myopathies, kyphoscoliosis, pectus excavatum, Duchenne muscular dystrophy, spinal muscular atrophies, myasthenia gravis, myotonic dystrophy, hypoplastic thorax syndromes, long-term noninvasive ventilation
Introduction
Since Lavoisier’s stipulation in 1791 that respiration is essentially a phenomenon of oxygen (O 2 ) consumption and carbon dioxide (CO 2 ) production, the chest wall has been recognized as the vital pump responsible for the movement of gases between the atmosphere and the lungs. Today, much is known regarding the adaptability of this vital pump to satisfy the changing metabolic needs under various physiological and pathological conditions (e.g., rest, exercise, and hyperthermia). Patients must overcome adverse conditions affecting chest wall function: conditions ranging from the cartilaginous, pliable rib cage of the preterm infant to the scoliotic thorax of the adolescent, and from the weak chest wall in neuromuscular disease to the stiff rib cage in asphyxiating thoracic dystrophy or obesity. The vital pump also participates in numerous functions, such as singing, talking, wind instrument playing, coughing, sneezing, load lifting, parturition, and hiccupping, all of which can interfere with lung ventilation.
In normal resting conditions, the diaphragm is the principal muscle used for inspiration while the accessory inspiratory muscles mainly stabilize the rib cage. When the inspiratory workload is increased, additional diaphragmatic motor units and accessory inspiratory muscles are recruited, the latter producing an upward motion of the ribs resulting in a more pronounced thoracic expansion. In normal resting conditions, expiratory muscles are inactive. During increased demands for air pumping, the expiratory muscles become activated during the second phase of expiration and decrease the end expiratory volume below functional residual capacity (FRC). Any decrease in the force of the expiratory muscles leads to an increased residual volume and decreased vital capacity.
Sleep is responsible for significant modifications in lung mechanics and respiratory muscle control. Assuming the supine position leads to a decrease in FRC, mainly due to the cephalad movement of the abdominal contents. Sleep also leads to a decrease in tonic activity of intercostal muscles and upper airway muscles, a phenomenon further pronounced in rapid-eye-movement (REM) sleep. These changes are responsible for paradoxical inward rib cage movement during inspiration in infants due to their compliant rib cage and in patients with neuromuscular disorders. Nocturnal hypoventilation with alteration of blood gases is often the first sign of chronic respiratory failure in progressive neuromuscular disorders, such as Duchenne muscular dystrophy. In addition to nocturnal hypoventilation, obstructive and/or central sleep-disordered breathing have been reported in a number of neuromuscular diseases, including Duchenne muscular dystrophy, myotonic dystrophy, congenital myopathies, metabolic myopathies, myasthenia gravis, and spinal muscular atrophy.
The Chest Wall in the Newborn
The features of the chest wall and respiratory muscles in the newborn period predispose to pump failure during illness ( Fig. 72.1 ). The very compliant chest wall of the premature and to a lesser extent the full-term infant results in chest wall distortion rather than efficient ventilation when inspiratory muscles contract against high mechanical loads, such as during lower respiratory infections. Ossification of the ribs is responsible for stiffening of the chest wall, so that chest wall compliance is up to seven-fold greater than lung compliance at birth, and reduced by half by the age of 3. The ribs are aligned perpendicular to the spine, rendering intercostal muscles unable to add volume with the “bucket handle” motion present in adults. This spine-rib angle approaches adult values by 2–3 years of age. The insertion of the diaphragm to the rib cage is also more perpendicular, which favors inward displacement of the lower ribs with any forceful diaphragmatic contraction. Therefore excessive diaphragm contraction has to be used to produce an adequate tidal volume. In addition, respiratory muscle mass and the proportion of fatigue-resistant fibers in the diaphragm are lower, resulting in less resistance to fatigue. This can be further amplified by nutritional difficulties and hypoglycemia, hypocalcemia, hypophosphatemia, or acidosis. To cope with all of this, the newborn in resting conditions breathes rapidly, with limited excursion of the diaphragm. In disease conditions, rather than increasing the depth of each breath, the newborn further increases the respiratory rate, thereby preventing diaphragmatic fatigue by decreasing the duration of inspiratory contraction. Functional residual capacity is actively maintained in the newborn by increasing the respiratory rate, hence decreasing the time devoted to lung deflation, or by breaking the expiratory flow through active glottal closure. Extremely prematurely born infants are particularly at risk of respiratory pump failure. Respiratory muscle fatigue is more often the cause of respiratory failure in the newborn than at any other time in life.

Respiratory Muscle Disorders—General Considerations
Diaphragm dysfunction can be due to a wide variety of disorders. Disorders of the diaphragm produce abnormalities in its position within the chest, excursion with inspiratory efforts, weakness and fatigability. These features often coexist. Hyperinflation due to intrathoracic airway obstruction flattens the diaphragm contour and reduces the surface area of the zone of apposition of the diaphragm, and hence inspiratory force. The higher the lung volume above FRC, the greater the reduction in maximal inspiratory force. Muscle weakness due to phrenic nerve injury leads to an elevated diaphragm on the affected side. Distortion of the diaphragm, as occurs with scoliosis, also causes a reduction in inspiratory force generation and excursion. Primary inspiratory muscle weakness predisposes these muscles to fatigue in the presence of mechanical loads due to disease processes.
Disorders that alter functional properties of the diaphragm and the other respiratory muscles include neuropathic processes, such as quadriplegia, or myopathic processes (e.g., muscular dystrophies). In addition, conditions producing primary respiratory disease can also affect the respiratory muscles secondarily. Examples include hyperinflation in asthma, cystic fibrosis, and bronchopulmonary dysplasia, as well as malnutrition, as observed with cystic fibrosis. Conditions that reduce blood flow to the diaphragm, such as heart failure and sepsis, predispose to diaphragm fatigue, as a less nutrient substrate is delivered to the respiratory muscles. Hypoxemia, as experienced at high altitude, reduces endurance time among healthy individuals breathing against large inspiratory resistive loads. Acute hypercapnia also produces inspiratory muscle fatigue in healthy adults breathing against increased mechanical loads.
In addition, certain therapies can lead to respiratory muscle dysfunction as a result of injury and atrophy. The use of paralytics, corticosteroids, and controlled mechanical ventilation leads to muscle atrophy remarkably quickly. Ventilator-induced diaphragm dysfunction can occur as quickly as 18 hours in patients without respiratory efforts receiving controlled mechanical ventilation. Diaphragmatic atrophy develops in proportion to the degree of muscle inactivity during controlled ventilator support, and the duration of that support. Promoting patient-initiated breaths while administering ventilator support prevents this effect. Aside from ventilator-induced diaphragmatic dysfunction, sepsis appears to result in very early onset muscle injury and loss of contractility before the occurrence of atrophy (i.e., independently of mechanical ventilation).
Clinically, these conditions can be present concurrently, such as controlled ventilation for paralyzed septic patients. Similarly, babies with bronchopulmonary dysplasia who are poorly nourished and who receive corticosteroids to treat acute viral lower respiratory tract infections have multiple etiologies for respiratory muscle dysfunction and failure. The relative importance of each factor when their combined risks occur clinically is unclear, although all etiologies for respiratory muscle dysfunction should be addressed.
Respiratory Muscle Fatigue
Respiratory muscle fatigue develops when there is a reduction in the force-generating capacity of the neuromuscular respiratory apparatus. This occurs when the energy supply to the respiratory muscles is reduced in the presence of increased work demands. It is also present when respiratory work requirements exceed the work capacity of normal respiratory muscles. In normal adults, inspiratory work that is less than 40% of maximal inspiratory force can be maintained indefinitely. The closer the workload approaches 100% of maximal inspiratory force, the faster respiratory muscle fatigue develops.
When a superimposed inspiratory mechanical load occurs, several mechanisms are called into play to meet muscle work requirements. There is an increase in respiratory drive and phrenic nerve output, which leads to the recruitment of diaphragm motor units. There is an increased recruitment of fast-twitch muscle fibers, which are more capable of generating force than slow-twitch (fatigue-resistant) fibers. There is also recruitment of accessory respiratory muscles of the chest wall and neck and a change in respiratory patterns to reduce the inspiratory time per breath. Despite these responses, mechanical loads imposed on the respiratory system can be of sufficient degree and duration to produce respiratory muscle fatigue and lead to hypoventilation and hypercapnia. Clinical features of impending acute respiratory failure are listed in Box 72.1 .
Inability to Perform Nonventilatory Functions
- ▪
Inability to eat/drink/cry
- ▪
Reluctance to pause breathing voluntarily
Major Use of Accessory Muscles
- ▪
Sternocleidomastoid muscles, pectoral
Increase in Respiratory Rate to Critical Level
- ▪
Adolescents: 55 breaths/min
- ▪
Children, 4–10 years: 70–75 breaths/min
- ▪
Infants, 1–3 years: 90 breaths/min
- ▪
Neonates: 120 breaths/min
Signs Reflecting Options Taken to Relieve Fatigue
- ▪
Shallow breathing
- ▪
Deep breaths with a brief pause to rest the muscles
- ▪
Respiratory alternans
Signs Indicating Pending Respiratory Arrest
- ▪
Cyanotic spells
- ▪
Cyanosis with brief cough or brief pause
- ▪
Recurrent apnea
- ▪
Sustained paradoxical thoracic/abdominal movement
- ▪
Drooling in absence of airway obstruction (cannot pause to swallow)
- ▪
Central nervous system signs (confusion)
Chronic respiratory muscle fatigue is less easily identifiable, and respiratory symptoms may not correlate well with the degree of respiratory muscle fatigue. General fatigue and dyspnea on exertion can be the first symptoms of chronic respiratory pump impairment. Additional manifestations of chronic respiratory muscle impairment include sleep hypoventilation, nocturnal parasomnias, enuresis, increased sweating, and daytime symptoms, such as morning headaches, hypersomnolence, chronic fatigue, and decreased attention span. New onset of unexplained symptoms of right heart failure due to unsuspected pulmonary hypertension may also reflect chronic sleep-related hypoxemia and hypoventilation. Serial assessment of chest wall function is necessary to predict or recognize the first signs of chronic respiratory muscle fatigue in patients with neuromuscular disorders ( Box 72.2 ).
Clinical Evaluation
- ▪
Chest wall configuration (e.g., scoliosis, overdistention)
- ▪
Pattern of spontaneous breathing
- ▪
Thoracic and/or abdominal breathing
- ▪
Paradoxical thoracoabdominal movements: inspiratory rib cage retraction, paradoxical inspiratory abdominal retraction (unilateral or bilateral)
- ▪
- ▪
Specific maneuvers:
- ▪
Maximal variation in thoracic circumference
- ▪
Maximal excursion of diaphragm (inspection and percussion)
- ▪
Cough strength?
- ▪
Laboratory Evaluation
- ▪
Roentgenograms:
- ▪
Cinefluoroscopic studies of diaphragmatic movements
- ▪
Plain roentgenograms in full expiration and inspiration
- ▪
- ▪
Real-time ultrasonography of the diaphragm
- ▪
Pulmonary function tests:
- ▪
Pressures: maximal inspiratory pressure (PI max) at mouth, sniff inspiratory pressure, maximal expiratory pressure (PE max) at mouth (transdiaphragmatic pressure)
- ▪
Peak cough flow
- ▪
Lung volumes, forced spirometry
- ▪
Variations/coordination of rib cage and abdominal diameter (respiratory inductance plethysmography)
- ▪
- ▪
Electromyographic studies of respiratory muscles (magnetic stimulation of phrenic nerve)
Etiologies
Chest wall movements should be envisioned as the result of a chain of effectors that successively transmit the drive to breathe from the respiratory centers to the upper motor neurons of the central nervous system, the lower motor neurons, and finally the respiratory muscle fibers. The central drive to breathe is transformed into a mechanical force resulting from contractions of respiratory muscles, which move the chest wall and generate inspiratory and expiratory pressures responsible for air movement into and out of the lungs. Table 72.1 illustrates the systemic conditions that cause disturbances at these various levels. Neurological diseases at the level of the central nervous system (upper motor neurons) or at the level of peripheral innervation of the chest wall (lower motor neurons) may lead to secondary dysfunction of the muscular components of the chest wall. The best examples of these entities are hemiplegia, quadriplegia, infantile spinal muscular atrophies, and polyradiculitis (Guillain-Barré syndrome). Myopathies from various types of muscular dystrophy or from metabolic origin, as well as disorders of the neuromuscular junction, result in failure of the respiratory muscles to produce an adequate contraction. In addition, systemic diseases associated with severe malnutrition may lead to a loss in respiratory muscle mass and force. Disorders involving the bony structures of the chest wall, such as scoliosis and asphyxiating thoracic dystrophy, are responsible for restrictive lung disease and impair the transformation of respiratory muscle contraction into adequate pressure, due to the malposition of these muscles. Finally, obesity causes an additional mechanical load on both the thoracic and abdominal components of the chest wall and limits its performance capacity.
Site of Defect | Causes |
---|---|
Central drive of breathing | Congenital or acquired |
Upper motor neuron | Hemiplegia |
Cerebral palsy | |
Quadriplegia | |
Lower motor neuron | Poliomyelitis |
Spinal muscular atrophies | |
Guillain-Barré syndrome | |
Tetanus | |
Traumatic nerve lesions | |
Phrenic nerve paralysis | |
Neuromuscular junction | Myasthenia gravis |
Congenital myasthenic syndromes | |
Botulism | |
Drugs | |
Respiratory muscles | Muscular dystrophies |
Congenital myopathies | |
Metabolic myopathies | |
Steroid myopathy | |
Connective tissue disease | |
Diaphragmatic malformation | |
Nonmuscular, chest wall structures | Scoliosis |
Congenital rib cage abnormality | |
Overinflated rib cage in chronic obstructive pulmonary disease | |
Connective tissue disease | |
Thoracic burns | |
Obesity | |
Giant exomphalos, Prune-belly syndrome |
Despite their differences in pathogenesis, the various entities that cause chest wall dysfunction share some clinical and physiological features. Chest wall dysfunction leads to restrictive pulmonary disease with reduced lung volume, reduced absolute flow rate values, normal forced expiratory volume in 1 second/forced vital capacity (FEV 1 /FVC) ratio, reduced strength of respiratory muscles and decreased ventilatory performance in response to exercise. Residual volume can be normal or augmented, depending on normal or decreased strength of the expiratory muscles, respectively. With respiratory muscle strength at less than 50% predicted, the decrease in vital capacity is generally greater than expected, due to the decreased compliance of the lung (atelectasis) and the rib cage (costovertebral and costosternal joint ankylosis). Hypercapnia is usually present during wakefulness when respiratory muscle strength is less than 25% predicted, but it can occur when weakness is less profound in the presence of coexisting mechanical loads due to additional respiratory disorders. With less severe disease, hypercapnia may be present during sleep, and patients are at risk of ventilatory failure during critical periods of their lives, such as in the neonatal period, during respiratory infections, following general anesthesia, and during the last trimester of pregnancy.
Pathogenesis
Diaphragm Paralysis
Diaphragm paralysis generally results from injury to the phrenic nerve during thoracic or neck surgery. Tumors of the mediastinum, peripheral neuropathy, and agenesis of the phrenic nerve are less likely causes. In the newborn, stretching of the root C3–C5 during breech delivery is a frequent cause, most often in association with brachial plexus injuries (Erb’s paralysis). Most diaphragm paralyses are on the right side, with bilateral paralysis occurring in only 10% of cases. Bilateral diaphragm paralysis can be responsible for a severe restrictive syndrome, with total lung capacity often below 50% of predicted value. Elevation of the paralyzed diaphragm is greater in the supine position, due to pressure from the abdominal content, and can be responsible for orthopnea.
Spinal Muscular Atrophies
Childhood spinal muscular atrophies (SMA) are a group of autosomal recessive neurodegenerative disorders with onset from birth to adulthood. Spinal muscular atrophies are due to a deficient survival motor neuron (SMN) protein, which is encoded by two adjacent and nearly homologous genes, SMN1 and SMN2, located on chromosome 5. SMN1 is the only gene able to produce the full-length SMN protein that is essential to the survival of motor neurons. Although the SMN2 gene differs by only a few nucleotides from the SMN1 gene, it mainly produces a truncated, rapidly degraded SMN protein. Spinal muscular atrophies are due to a mutation of the SMN1 gene, which results in the degeneration of the anterior horn cells of the spinal cord and often of the bulbar motor nuclei, leading, in turn, to skeletal muscle weakness and atrophy.
Neuromuscular Junction Diseases
Myasthenia Gravis
Juvenile Myasthenia Gravis.
Similar to the adult form, juvenile myasthenia gravis is an acquired autoimmune disorder of neuromuscular transmission associated with circulating autoantibodies against acetylcholine receptors (AChR) in 80%–90% of cases. The juvenile form accounts for 10% of all cases of myasthenia gravis.
Neonatal Myasthenia Gravis.
Neonatal myasthenia is a transient disorder, which affects 10%–30% of infants born to mothers with autoimmune myasthenia gravis. It results, at least partly, from passively transmitted maternal AChR autoantibodies, even if the mother has shown no clinical symptoms.
Congenital Myasthenic Syndromes
Congenital myasthenic syndromes refer to a group of various inherited disorders resulting from mutations of genes crucial for neuromuscular transmission. At least 24 different genes are known to cause congenital myasthenic syndromes.
Myopathies
Duchenne Muscular Dystrophy
Duchenne muscular dystrophy (DMD) is the most common and severe form of muscular dystrophy afflicting humans. Inherited as an X-linked recessive trait, it has an incidence of approximately 1 : 3500 male births. Almost one-third of cases result from new mutations. The gene responsible has been localized within the dystrophin gene located on the short arm of the X chromosome (Xp21). Dystrophin links the normal contractile apparatus to the sarcolemma in skeletal muscle. Dystrophin gene mutations result in either a lack of dystrophin (usually DMD) or the expression of mutant forms of dystrophin (usually Becker muscular dystrophy).
Other Myopathies
Aside from DMD, myotonic dystrophy is the other muscular dystrophy which commonly leads to respiratory failure before adulthood. Myotonic dystrophy type I (Steinert disease) is a multisystem disease, which includes anomalies of the skeletal and smooth muscle as well as of the eye, central nervous system, heart, and endocrine system. Myotonic dystrophy type I follows a pattern of autosomal dominant inheritance and has a worldwide prevalence of 1 : 8000. The culprit mutation is an expansion of the cytosine-thymine-guanine (CTG) trinucleotide in the nontranslating region of the dystrophia myotonica protein kinase (DMPK) gene on chromosome 19. The highly variable phenotype, with an age of onset spanning from the prenatal period to adulthood, is closely related to the size of the CTG expansion, so that a longer expansion is responsible for a more severe phenotype.
In addition, pulmonary function abnormalities and respiratory failure have been reported in metabolic myopathies as well as in the childhood form of limb girdle muscle dystrophy, facioscapulohumeral dystrophy, and Emery-Dreifuss syndrome.
Disorders Involving the Bony Structure of the Chest Wall
Pectus Excavatum
Pectus excavatum is characterized by a symmetric or asymmetric depression of the sternum and anterior chest deemed to be due to defects in the sternum cartilage or overgrowth of the costal cartilage of the ribs. It is accompanied by an abnormally shallow chest in the anterior-posterior dimension in 60% of cases, described as a pectus gracilis. Severity of the pectus excavatum is directly correlated with the severity of the pectus gracilis, raising the question as to whether the pectus excavatum is a thoracic as well as a sternal deformity. It occurs predominantly in white males, with an estimated incidence of 1 in every 300–400 live births and represents more than 90% of all congenital chest wall abnormalities. Its etiology remains unclear, although a genetic base is suggested by the observation that about one-fourth of patients have an affected family member. Pectus excavatum appears to be polygenetic, following various patterns of inheritance. It is frequently associated with idiopathic scoliosis, and is a component of a number of connective tissue disorders, especially Marfan syndrome. Severe pectus excavatum can induce mild restrictive syndrome and can be associated with limitation in exercise tolerance.
Kyphoscoliosis
Scoliosis is the result of underlying pathological processes leading to lateral displacement in the coronal plane of the spine. Kyphosis describes the curvature of the spine in the sagittal plane, which curves the spine forward. There are children with major scoliosis and minor kyphosis and children with major kyphosis and minor scoliosis. Kyphoscoliosis is also associated with rotation of the spine, which contributes to spine deformity and distorts the thoracic cage. Kyphoscoliosis distorts the ribs, sternum, and respiratory muscles, as well as intrathoracic organ orientation.
Classification of Kyphoscoliosis.
The age of onset and etiology are most often used to classify kyphoscoliosis in children. The most common form of scoliosis has its onset at the time of adolescence in otherwise healthy children. Less than 10% of spine deformities are those first diagnosed before 10 years of age, known as early onset scoliosis. The specific ages of different idiopathic scoliosis and the categories of etiologies that are nonidiopathic are listed in Box 72.3 .
Idiopathic (85% of All Scoliosis)
- ▪
Infantile (<3 years old)
- ▪
Juvenile (3–9 years old)
- ▪
Adolescent (10 years old to skeletal maturity)
Congenital (5% of All Scoliosis)
- ▪
Failure of formation (hemivertebra(e))
- ▪
Failure of segmentation
- ▪
Mixed
Neuromuscular (5% of All Scoliosis)
- ▪
Neuropathic
- ▪
Lower motor neuron (e.g., poliomyelitis)
- ▪
Upper motor neuron (e.g., cerebral palsy)
- ▪
Other (e.g., syringomyelia)
- ▪
Myopathic
- ▪
Progressive (e.g., muscular dystrophy)
- ▪
Static (e.g., amyotonia)
- ▪
Others (e.g., Friedreich’s ataxia)
Associated With Neurofibromatosis (Von Recklinghausen Disease)
Mesenchymal
- ▪
Congenital (e.g., Marfan syndrome)
- ▪
Acquired (e.g., rheumatoid disease)
- ▪
Others (e.g., juvenile apophysitis)
Traumatic
- ▪
Vertebral (e.g., fracture, radiation, surgery)
- ▪
Extravertebral (e.g., burns, thoracoplasty)
- ▪
Secondary to irritative phenomenon (e.g., spinal cord tumor)
Idiopathic Kyphoscoliosis.
Idiopathic kyphoscoliosis is defined as a spine deformity without any overt malformations of the vertebral bodies or predisposing conditions leading to secondary spine deformity. Autosomal dominant, X-linked and multifactorial patterns of inheritance have been reported to account for the hereditary factors of the disease. Idiopathic scoliosis, which accounts for 80%–85% of cases, is further categorized into infantile, juvenile, and adolescent idiopathic scoliosis, depending on the age of onset.
Congenital Scoliosis.
Congenital scoliosis is due to the presence of vertebral anomalies with or without associated rib deformities. Common malformations include single or multiple hemivertebrae and segmentation defects, which produce block vertebrae of variable length. Congenital scoliosis can be present at birth with associated pulmonary hypoplasia, or can be mild and diagnosed later in childhood. Congenital scoliosis is often accompanied by other congenital anomalies and represents one component of a multiorgan syndrome (e.g., VACTERL syndrome).
Respiratory Effects of Kyphoscoliosis.
The respiratory effects of kyphoscoliosis vary with age of onset, initial severity, and progression of deformity over time. The degree of respiratory impairment is primarily due to the constraint of the lung by a relatively rigid thoracic cage related to the severity of the three-dimensional deformity of the spine. In addition, kyphoscoliosis can be responsible for a decrease in inspiratory muscle strength, as well as impaired lung growth.
The consequences of scoliosis on lung growth remain unclear. Postmortem quantitative studies among adolescents who died of severe kyphoscoliosis suggest that alveolar number and complexity are decreased in congenital or infantile scoliosis, whereas the alveoli may not enlarge normally in idiopathic juvenile or adolescent scoliosis. The number of pulmonary vessels in scoliotic children is also reduced in proportion to alveolar hypoplasia. Lung function studies have demonstrated a greater reduction in vital capacity for the same Cobb angle among children with early onset scoliosis compared to adolescent onset, raising the question of associated pulmonary hypoplasia in those with early onset spine disease. Pulmonary hypoplasia has recently been produced in rabbits that undergo iatrogenic scoliosis with postnatal rib tethering. Pulmonary hypertension and cor pulmonale have been described in a small minority of children with spine and chest deformities, and this number may be even less when sleep-related breathing abnormalities are treated with oxygen or noninvasive positive pressure ventilation.
The term “thoracic insufficiency syndrome” has been used to identify those children with spine and chest wall disorders that impair lung function, or postnatal lung growth with skeletal immaturity and future bone growth. The syndrome includes children with early onset scoliosis, regardless of etiology, which is sufficiently severe to impair lung function. Early onset scoliosis may become thoracic insufficiency syndrome as it progresses and as respiratory function becomes compromised over time. Clinical and/or objective measurements of lung function are needed to make this diagnosis.
Hypoplastic Thorax Syndromes
A variety of syndromes are associated with small thoraces leading to secondary respiratory failure in the first year of life and high mortality rates. These conditions represent a separate category of thoracic insufficiency syndrome. Hypoplasia can occur due to a reduced circumference or due to reduced thoracic vertebral height. There is a continuum of hypoplasia in most of these syndromes, so some patients live to adulthood without respiratory support.
Asphyxiating Thoracic Dystrophy.
The most common hypoplastic thorax syndrome is asphyxiating thoracic dystrophy, also known as Jeune syndrome or thoracic-pelvic-phalangeal dystrophy. This autosomal recessive disorder is part of the ciliary chondrodysplasias, a group of inherited conditions resulting from cilia malfunction affecting skeletal development in mammals. It is characterized by a narrow, hypoplastic rib cage, and generalized chondrodystrophy with short limb dwarfism. Pelvic and phalangeal abnormalities, polydactyly, renal, and hepatic disorders, thrombocytopenia as well as Shwachman syndrome have also been reported in conjunction with asphyxiating thoracic dystrophy, with renal disease emerging later in life as a serious concern. Estimates suggest that up to 600 patients per year are born with this disease worldwide.
Jarcho-Levin Syndrome.
An alternative type of chest wall hypoplasia is illustrated by patients with Jarcho-Levin syndrome. This syndrome includes two distinct genetic disorders, spondylocostal dysostosis and spondylothoracic dysplasia, which present with multiple vertebral and costal anomalies. The vertebral anomalies result in a shortened spinal height and increased anterior–posterior dimension albeit with a reduced intrathoracic volume.
Spondylocostal dysostosis is associated with mutations in the DLL3 (delta-like canonical Notch ligand 3) gene, which is involved with the Notch pathway in separating future vertebrae from one another during early development (somite segmentation). It has both autosomal dominant and recessive inheritance patterns. Spondylothoracic dysplasia is associated with mutations in the MESP2 (mesoderm posterior bHLH transcription factor 2) gene, which is of Puerto Rican heritage and controls activity of genes in the Notch pathway. It is inherited in an autosomal recessive manner.
Clinical Features
Diaphragm Paralysis
Unilateral diaphragm paralysis is usually asymptomatic, but can lead to exercise limitation in some patients. When bilateral, the prominent clinical features include unexplained tachypnea, hypoxia, or respiratory distress, which usually worsen in the supine position. Respiratory failure or difficult weaning from ventilatory support after surgery can occur, especially in the neonate or when underlying pulmonary disease is present. In chronic cases, respiratory insufficiency, recurrent atelectasis and infections can be observed, as well as failure to thrive.
Spinal Muscular Atrophies
While there is no clear delineation of SMA subtypes, the clinical classification by age of onset and maximum function achieved is useful for prognosis and management.
Spinal Muscular Atrophy Type I
In infants with SMA I (incidence ~1 : 10,000 live births), poor muscle tone and muscle weakness begin at age 0–6 months and are associated with a lack of motor development and an inability to sit without support. There is minimal or absent facial weakness, but fasciculation of the tongue is observed in most children. Tendon reflexes are absent. There is no sensory loss and cerebral function is normal, including intellect. A prenatal subtype with overt manifestations at birth occurs. Infants with prenatal SMA and SMA I have impaired head control, with a weak cry and cough. Intercostal muscle weakness is responsible for paradoxical inward rib cage movement with each inspiration, the diaphragm being relatively spared. Chest x-rays show a reduced intrathoracic volume and a bell-shaped thorax. Affected patients suffer from swallowing disorders and aspiration, and are at risk of recurrent respiratory failure with viral respiratory infections. The prognosis is poor, with 95% of infants dying from respiratory failure by the age of 18 months without intensive respiratory intervention.
Spinal Muscular Atrophy Type II
In children with SMA II, muscle weakness usually begins after the age of 6 months. The child can sit independently when placed in a sitting position, but cannot walk. Low muscle tone is present and tendon reflexes are absent in about two-thirds of children. They have average intellectual skills during the formative years and above average by adolescence. Swallowing disorders, gastric reflux, recurrent atelectasis, and respiratory infections vary in their degree of severity and carry the risk of respiratory failure. In the majority of older children, thoracic and thoracolumbar scoliosis further aggravates respiratory function. Even after the spine is fused and stabilized, the chest wall can continue to collapse, producing the appearance of a closing parasol ( Fig. 72.2 ). Typically, recurrent pulmonary infections precede nocturnal hypoxemia, nocturnal hypoventilation, and then daytime hypoventilation. With adequate respiratory management tailored to respiratory involvement, including noninvasive ventilation, mechanical in-exsufflation, and the prevention of respiratory infections, a good quality of life and lifespan into adulthood is expected for most patients.
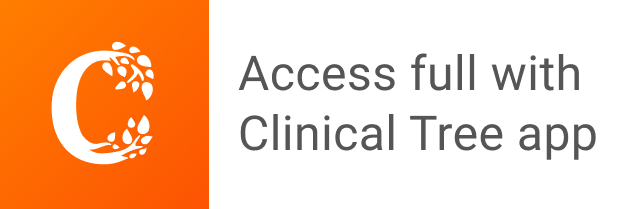