Outline
The Postnatal Heart is Not a Postmitotic Organ, 43
Sources of Controversy in Postnatal Mammalian Cardiomyocyte Proliferation 44
Evidence of Cardiomyocyte Turnover in the Adult Heart, 44
Molecular Basis of the Terminally Differentiated State of Cardiomyocytes 45
Mechanisms of Cardiac Regeneration, 51
Endogenous Heart Regeneration in Lower Vertebrates and Mammals, 51
Induction of Cardiomyocyte Proliferation, 51
Cyclins and Cyclin-Dependent Kinases, 51
microRNAs, 51
Transcription Factors, 52
Nerves, 53
Hippo Signaling, 53
Ventricular Unloading, 54
Exogenous Cardiomyocytes for Cell Therapy, 56
Conclusions and Future Directions, 61
Cardiac regeneration refers to the process of heart repair after an injury through repopulation of specific cell types to replace the damaged tissue, to regain functional capacity. Although credible evidence existed for this phenomenon in lower vertebrates, the belief in the potential for cardiac regeneration in mammals is a recent development. It was previously believed that the heart is a “postmitotic” organ whose workhorse cell, the cardiomyocyte, is incapable of cell division. In the past two decades, the heart’s reputation dramatically changed from a postmitotic organ to a highly proliferative one and, more recently, to an organ with only modest turnover capacity. These opposing views of cellular turnover in the mammalian heart generated a number of exciting and precise studies that provide significant and novel insights into heart regeneration. Indeed, we now have stringent evidence that the adult mammal heart is capable of a low rate of turnover throughout life, with a notable window for complete regenerative potential restricted to the early neonatal period. In this chapter, we will describe the physiologic and pathologic cardiomyogenesis that occurs in mammals, and we will discuss different classes of investigative therapies with the potential to stimulate cardiac regeneration in the adult heart.
The Postnatal Heart is Not a Postmitotic Organ
The foundations for the notion that the heart is a static organ incapable of regeneration were established in the mid-1920s. A significant publication in 1925 claimed that mitotic figures are not detectable in human cardiomyocytes, which thereby were considered cells irreversibly withdrawn from the cell cycle: this study effectively introduced the concept that the heart is a terminally differentiated, postmitotic organ.
The notion that the pool of myocytes present at birth is irreplaceable during the life span of the organism gained further support from a series of autoradiographic studies conducted in the 1960s that delivered tritiated thymidine to the myocardium during postnatal development and pathologic overload to quantify DNA replication. The degree of DNA synthesis in myocyte nuclei was negligible, and therefore the increase in heart size that occurs with age was correlated to cardiomyocyte cell size growth from increased cell volume (i.e., hypertrophy rather than hyperplasia was thought to be the predominant mechanism for the postnatal gain in heart weight).
In the late 1990s and early 2000s, a highly publicized series of publications from the Piero Anversa group directly challenged the dogma of the heart as a postmitotic organ. They evaluated hearts from patients who died a few days after a myocardial infarction (MI) (as well as control hearts), and they observed expression of Ki-67, a cell cycle–associated nuclear antigen, in the border zone of the infarct and the remote zones. They found putative evidence of mitotic spindles, formation of contractile rings, karyokinesis, and cytokinesis in tissue sections by immunofluorescence in injured hearts. From these observations, they concluded that there is significant cardiomyogenic potential in the human heart and that “regeneration of myocytes may be a critical component of the increase in muscle mass of the myocardium.” This report spurred intense investigation into further characterization of postnatal cardiac growth and raised important questions about the parent cell of origin of new cardiomyocytes. However, another study raised doubts regarding the use of Ki-67 to prove cell division, because it showed that Ki-67 expression after human MI may be due to polyploidization rather than bona fide mitosis.
In subsequent reports the Anversa group provocatively claimed that on average 22% new myocytes were generated every year in humans, suggestive of a life span of 4.5 years for this cell type. Independent efforts to replicate these results using different techniques could not corroborate this degree of cardiomyocyte turnover. In the past few years, clonal analysis and radioisotope labeling methods have provided the most reliable data for cardiac growth and its dynamics after birth in mammals. It is now believed that the mammalian heart experiences a very low—albeit not zero—rate of turnover mediated by preexisting cardiomyocytes, with a possible small increase in cardiomyogenesis after MI which has tangential clinical impact.
Sources of Controversy in Postnatal Mammalian Cardiomyocyte Proliferation
To understand the basis for conflicting results and the wide range of myocyte proliferation rates in the field, it is important to understand the cardiomyocyte cell cycle. The fundamental basis for controversy in the field is the uncoupling of DNA synthesis, nuclear division (karyokinesis), and abscission (cytokinesis)—leading to multinucleated and polyploid cells—that occurs in mammalian cardiomyocytes, which confounds interpretation of traditional cell turnover assays based on DNA replication.
Nearly 20 years ago, the administration of thymidine analogues to mice revealed two temporally distinct phases of DNA synthesis that characterize the prenatal and early postnatal growth of the myocyte compartment. The first peak in DNA synthesis was observed in the fetal heart and attributed to cardiomyocyte proliferation. Conversely, the second phase of DNA synthesis, which was detected 4 days after birth, primarily occurs due to binucleation without cytokinesis. These fundamental findings have been reproduced numerous times using other methodologies to identify replicating nuclei in cardiomyocytes in rodents ( Fig. 3.1 ). These findings also illuminate the challenge of measuring cardiomyocyte turnover: not all DNA synthesis results in cytokinesis. In fact, there is a progressive decline in the rate of cardiomyocyte cell proliferation concomitantly with a rise in DNA synthesis. In mice, around postnatal day 7 (P7) there is a peak in cardiomyocyte binucleation and around P14 there is a peak in polyploidy (DNA replication without nuclear division), leading to 4n, 8n, 16n, etc. chromosome copy numbers. These consistent results cast a penumbra of uncertainty over any method that relies exclusively on DNA or nuclear division as a proxy for cell division in cardiomyocytes. In addition, markers of S phase of the cell cycle may be incorporated during DNA repair, leading to a false-positive interpretation. Ultimately, there is no marker of mitosis that prima facie confirms that cytokinesis has occurred/will occur: even cytokinesis-associated proteins may moonlight in other phases of the cell cycle, thus limiting their utility to specifically denote abscission.

Another factor that complicates interpretation of proliferation assays in cardiomyocytes is the difficulty to distinguish cardiomyocyte/cardiomyocyte nuclei from nonmyocyte cell types in tissue sections histologically. Sophisticated methodologies like lineage tracing and fate-mapping mouse models have provided significant insights into postnatal cardiomyocyte turnover, but they carry their own shortcomings such as questions about promoter fidelity, inefficient reporter gene expression, and cellular fusion or transfer of reporter proteins.
Collectively, these considerable caveats impede use of routine assays to confirm or quantify cardiomyocyte mitosis. As such, it became imperative for the field of cardiovascular biology to turn to more rigorous techniques to prove occurrence of new cardiomyocyte generation, such as the use of multiple mitotic markers and analysis of cell size and cell number quantification within the same study. In addition, the field has increasingly relied on clonal analysis because it enables precise determination of parent-progeny relationships and relies on completion of cytokinesis. However, this highly rigorous approach also carries many of the fate-mapping caveats outlined earlier. Finally, radioisotope labeling–based methods combined with sophisticated mathematical modeling in mice and in humans buttress the clonal analysis findings and have provided us the most meticulous calculations of the rate of cardiac growth in mammals.
Evidence of Cardiomyocyte Turnover in the Adult Heart
Frisen and coworkers used a carbon-14 ( 14 C)-based methodology to calculate the precise rate of cardiomyocyte turnover in the human heart in 2009. In brief, they obtained autopsied human heart samples and isolated cardiomyocyte nuclei using specific antibodies. Then they retrospectively determined the birth date of cardiomyocytes by measuring and correlating 14 C levels in cardiomyocyte genomic DNA with atmospheric 14 C levels. The characteristic exponential decay of atmospheric 14 C after the 1963 Limited Nuclear Test Ban Treaty allowed them to ascribe a date mark to each cardiomyocyte. They operated on key assumptions that the 14 C level in a cell’s DNA remains stable after its last cell division and reflects the concentration of 14 C in the atmosphere at the time of last cell division. Thus the rise and decay of 14 C in the Earth’s atmosphere due to historical nuclear weapons testing facilitated precise calculations of cardiomyocyte turnover kinetics in humans.
These 14 C radioisotope label measurements convincingly demonstrated that the heart indeed is not a postmitotic organ. Frisen and colleagues showed that the annual birth rate of cardiomyocytes in humans is highest in the first decade and after that decreases to less than 1% throughout life. In fact, the pattern is very similar to that observed in mice (see Fig. 3.1 B ). Due to differing interpretations of the 14 C data, Frisen and coworkers performed additional stereological analysis to further validate their results. They obtained tissue from 29 human postmortem samples with no history or evidence of heart disease and measured the proliferation kinetics of cardiomyocytes, endothelial cells, and mesenchymal cells in the heart. Due to the use of stereology, they were also able to measure total cell numbers and cell volumes in a given tissue sample. They confirmed that the cardiomyocyte cell volume increases with age ( Fig. 3.2E ) but the total number of cardiomyocytes remains relatively constant (see Fig. 3.2B ). After modeling for binucleation and ploidy that occurs in the first three decades of life ( Fig. 3.3A ; also see Fig. 3.2C ), they confirmed their initial findings that the cardiomyocyte proliferation rate in humans is less than 1% after age 10, and this rate continues to decline with aging (see Fig. 3.3 C ). They further investigated proliferation rates in specific regions of the heart and found no differences in cardiomyocyte renewal kinetics among the myocardial layers (e.g., endocardium, myocardium, epicardium), zones (e.g., apex, base), or chambers, arguing against the existence of a region-specific progenitor population.


Senyo and colleagues extended the aforementioned findings to mice by combining genetic fate-mapping with multi-isotope imaging mass spectrometry (MIMS), which requires administration of [ 15 N]thymidine and is analogous to the 14 C radiolabel technique ( Fig. 3.4 ). They calculated an annual rate of turnover of murine cardiomyocytes from preexisting cardiomyocytes at 0.76%. They also found that 8 weeks after an MI in adult mice, 3.2% of the cardiomyocytes in the infarct border zone had undergone division. However, as with the work of Frisen and coworkers, certain assumptions have to be made about the fraction of DNA synthesis that occurs due to multinucleation and polyploidy as opposed to completion of the cell cycle through abscission. For example, only mononuclear diploid cardiomyocytes were counted as true newborn daughter cells, whereas polyploid or multinucleate cardiomyocytes with nuclear 15 N were presumed to be the result of DNA synthesis without cytokinesis.

Although these radioisotope labeling–based analytical methods provided meticulous insights into cardiomyocyte renewal dynamics that corroborated across mammalian species, these techniques could not prove the ontogeny of postnatal cardiomyogenesis at the single cell level. Because the source of new cardiomyocytes was a key question in the field, clonal and nonclonal fate-mapping techniques were used to definitively provide an answer.
The “Mosaic Analysis of Double Markers” (MADM) model is ideally suited to the study of the heart because Cre recombination in replicating cells can result in asymmetric inheritance of fluorescent labels in the two daughter cells: one nucleus obtains the tdTomato gene and the other nucleus receives the green fluorescent protein (GFP) gene. If there is no nuclear division or no cytokinesis, then the parent cell will remain double-positive for the two fluorescent proteins. However, if the dividing parent cell undergoes mitosis with cytokinesis, the two daughter cells will become asymmetrically fluorescent ( Fig. 3.5A ). Thus cytokinesis is necessary and sufficient to generate single-labeled cells, and the sibling relationship of daughter cells can be ascertained due to the asymmetric label. Moreover, because the labeling is indelible, MADM permits clonal analysis of daughter cells (i.e., assessment of the proliferative capacity of individual cells over time).

Using MADM, Ali and coworkers directly showed that existing cardiomyocytes that express the sarcomeric protein α-myosin heavy chain are the parent cells of postnatal newborn cardiomyocytes (see Fig. 3.5 ). Cardiomyocytes that underwent division appeared to divide only once over the time course analyzed, arguing against a small pool of highly proliferative cardiomyocytes. Moreover, all sibling daughter cells were both cardiomyocytes; there were no instances of nonmyocyte and myocyte sibling cells, arguing against the existence of a bipotent or multipotent cardiac progenitor. However, a shortcoming of MADM is that, owing to inefficient Cre recombination, it underestimates the rate of cardiomyocyte proliferation and cannot directly dis prove the existence of a progenitor parent cell. Nonetheless, other lineage tracing models estimate the contribution to cardiomyogenesis from resident stem/progenitor cells at less than 0.01% per year.
These prior studies left open the question as to whether all cardiomyocytes are able to participate in postnatal cardiomyogenesis or whether a unique subset of cardiomyocytes boasts this potential. Based on their findings that exposure to normoxia after fetal life is associated with postnatal cardiomyocyte cell cycle arrest, Kimura and colleagues developed a novel hypoxia fate-mapping model to ascertain the mitotic potential of hypoxic cardiomyocytes ( Fig. 3.6 ). They showed that hypoxic cardiomyocytes, as marked by Hif-1α expression, comprise the predominant subtype of adult cardiomyocytes that undergoes postnatal division during physiologic aging; these cells also proliferate after an MI injury. The majority of newborn cardiomyocytes—as identified by nuclear BrdU incorporation—appear to arise from this cohort of hypoxic cardiomyocytes, which are smaller, predominantly mononuclear, and accumulate less oxidative DNA damage than the remaining cardiomyocytes. These findings strengthen the claim that the majority of mammalian adult cardiomyocytes are postmitotic and denote hypoxia signaling as an important player in postnatal cardiomyogenesis.

The combination of lineage tracing, radioisotope labeling, and clonal analysis has provided the most meticulous assessments of both cardiomyocytes renewal rates and ontogeny in the mammalian heart. These studies clearly demonstrate that, although the majority of mammalian adult cardiomyocytes do not participate in cell division during aging or with injury, a small subset retains mitotic potential that may be targeted for therapeutic purposes.
Molecular Basis of the Terminally Differentiated State of Cardiomyocytes
Evidence from animal models showed that only a very small fraction of cardiomyocytes undergoes turnover in the mammalian heart, whereas the remainder appears to be cell cycle arrested. The search for the molecular control underlying withdrawal of most postnatal cardiomyocytes from the cell cycle resulted in the compilation of a list of genes that are known to promote growth arrest in multiple systems. Cessation of myocyte proliferation at birth has been linked to the downregulation of cyclins, cyclin-dependent kinases (CDKs), and E2F transcription factors and to the upregulation of the negative modulators of cell cycle progression: Cdkn1a, Cdkn1b, Cdkn1c, and Cdkn2c. The presence of functional growth factor receptors on the plasma membrane of mature myocytes endows them with the capacity for physiologic and/or pathologic hypertrophy needed in response to pathologic loads in combination with reexpression of the fetal gene program.
Based on observations in skeletal myoblasts, it was hypothesized that the retinoblastoma (Rb) protein permanently inhibits the transition of cardiomyocytes from the G 1 to the S phase of the cell cycle. For example, the neonatal heart harbors inactive (hyperphosphorylated) Rb, coincident with myocyte renewal, whereas adult and hypertrophied hearts are characterized by high levels of hypophosphorylated, active Rb, which opposes myocyte division. Similarly, the cell cycle regulators cyclins A, D1, D2, and D3 are highly expressed in the developing heart and rapidly downregulate after birth.
Recent evidence supports the hypothesis that activation of the DNA damage response (DDR) pathway in the postnatal period leads to cell cycle arrest in mammalian cardiomyocytes. The relative hypoxemia of fetal circulation is replaced with normoxia after birth upon exposure to environmental oxygen. Concomitant with this rise in circulating oxygen tension is a transition in the neonatal mouse heart from glycolysis to aerobic metabolism, which is a metabolic switch that leads to reliance on mitochondria for energy generation and therefore leads to high levels of reactive oxygen species (ROS). Puente and colleagues showed that high levels of ROS in the postnatal heart generates oxidative DNA damage, which activates the DDR pathway. They showed that exposure to postnatal hypoxemia, inhibition of DDR, and ROS attenuation extend the postnatal window of cardiomyocyte proliferation. On the other hand, hyperoxemia and ROS generators shorten the window. In further support of this hypothesis, Nakada and colleagues were able to partially ameliorate the postmitotic phenotype of adult cardiomyocytes by exposing mice to environmental hypoxia. They showed that there is increased proliferation of cardiomyocytes at 7% atmospheric oxygen level due to a decrease in ROS and oxidative DNA, compared with normoxic control mice that experience 21% environmental oxygen ( Fig. 3.7 ).

The polyploidization of mammalian cardiomyocytes has also been associated with the loss of proliferative potential, although polyploid liver cells are capable of division and contribute to hepatocyte regeneration. A recent comprehensive survey of the cardiomyocyte nucleation status in 120 inbred mouse strains found a wide variation in the frequency of mononuclear diploid cardiomyocytes, ranging from 2.3% to 17.0% of all cardiomyocytes. Intriguingly, it was observed that strains with more mononuclear diploid cardiomyocytes had increased cardiomyocyte proliferation and regeneration after adult MI injury. This hypothesis was directly tested in zebrafish, in which 99% of adult cardiomyocytes are mononuclear diploid and retain the capacity to regenerate after injury. Transient gene perturbation resulted in polyploidy in half of the zebrafish cardiomyocytes (upon activation of a dominant-negative version of a key cytokinesis protein, ECT2). In this model, mononuclear diploid cardiomyocytes proliferated at higher rates than polyploid cardiomyocytes after amputation of the ventricular apex in adult zebrafish. Intriguingly, when the fraction of polyploid cells rises to approximately 50% of the entire cardiomyocyte cohort, the regenerative ability of adult zebrafish was significantly impaired. It remains to be seen whether such a mechanism applies also to the mammalian heart. Moreover, whether binuclear and polyploid mammalian cardiomyocytes may undergo abscission during aging or after a pathologic insult remains an open question.
Another challenge to the ability of postnatal cardiomyocytes to divide may lie outside the cell: a recent report documented a change in extracellular matrix composition in the first week of life in neonatal mice that correlates with the loss of significant proliferative capacity. Specifically, the extracellular matrix proteoglycan agrin is present in neonatal mouse hearts and is necessary for neonatal cardiac regeneration. Agrin expression is lost in the first week of life, but a single myocardial injection of agrin after MI in adult mice increased cardiomyocyte proliferation, reduced fibrosis, and improved systolic function.
The mechanism by which the extracellular matrix plays in a role in cardiomyocyte proliferation could be through transduction of mechanical load, which has been shown to influence the cardiomyocyte nuclear and mitochondrial state in the human heart. A natural model for unloading the left ventricle is the left ventricular assist device (LVAD), which is implanted in advanced heart failure patients as a bridge to orthotopic heart transplant or as a destination therapy. LVADs are mechanical pumps that increase cardiac output and systemic perfusion and thereby improve end-organ function. Notably, a small subset of LVAD patients has experienced sufficient myocardial recovery to be able to undergo LVAD explantation. The functional recovery in these patients suggests a salutary effect of pressure unloading of the left ventricle as accomplished by an LVAD. Cardiac biopsies from LVAD patients showed a decrease in global cardiomyocyte polyploidy (which is increased in failing hearts) and an increase in the number of diploid cardiomyocytes, which suggests that mitosis of cardiomyocytes and/or abscission of polyploid cardiomyocytes may be occurring in unloaded hearts. Moreover, post-LVAD hearts have reduced mitochondrial DNA copy number, smaller cardiomyocytes, and reduced activity in the DDR pathway. Consistent with prior findings that activation of the DDR pathway dampens cardiomyocyte division, post-LVAD hearts have increased expression of mitosis- and cytokinesis-associated proteins ( Fig. 3.8 ). These findings imply that mechanical unloading may lead to cardiomyocyte cell division and thus may represent another means to achieve cardiac regeneration.

Viewed together, these studies demonstrate that a number of cell autonomous as well as non–cell autonomous mechanisms underlie the terminally differentiated state that characterizes the majority of cardiomyocytes in the mammalian heart. It remains unclear at this time if all the previously described mechanisms apply to all cardiomyocytes or whether subpopulations of cardiomyocytes are differentially affected. Further evaluation and manipulation of these pathways will be necessary to optimize therapies that undergird cardiac regeneration.
Mechanisms of Cardiac Regeneration
Endogenous Heart Regeneration in Lower Vertebrates and Mammals
There are few known species with the capacity to substantially regenerate the heart. In 2002 Poss and colleagues first demonstrated that surgical resection of 20% of the zebrafish single ventricle was followed by full regeneration without fibrotic scarring within 60 days ( Fig. 3.9 ). Fate mapping confirmed that cardiac regeneration is primarily mediated by proliferation of preexisting cardiomyocytes in adult zebrafish.

The demonstration that vertebrates like teleost fish could regenerate the heart raised the exciting possibility that mammals may also possess this capability. In 2011 Sadek and colleagues adapted the zebrafish ventricular apical resection model to neonatal mice to assess for cardiac regeneration. This landmark study revealed that 1 day postpartum (P1) mice retain a capacity to regenerate their hearts following cardiac resection ( Fig. 3.10 ). P1 mice underwent surgical resection of 15% of the left ventricle, and within 21 days the myocardium was completely replaced, with normalization of the left ventricular ejection fraction at 2 months after resection. Genetic fate mapping suggested that the primary source of regeneration is expansion of existing cardiomyocytes. Notably, apical resection did not result in cardiomyocyte hypertrophy or significant fibrosis.
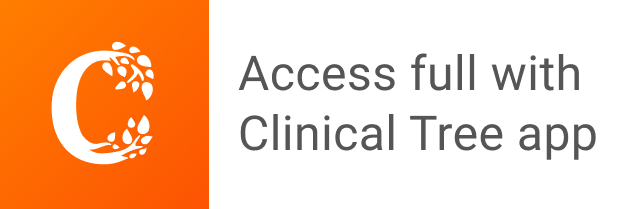