Outline
Contractile Dysfunction, 30
Normal Excitation-Contraction Coupling, 31
Impaired Ca 2+ Handling in Failing Cardiac Myocytes, 31
Reduced SR Ca 2+ Reuptake in Heart Failure, 32
Increased NCX Activity in the Failing Heart, 32
“Leaky” RyR2 Cause Diastolic SR Ca 2+ Loss in Heart Failure, 33
Contribution of Impaired Ca 2+ Handling to Arrhythmia, 34
Sarcomeric Dysfunction in Heart Failure, 34
Global Mechanisms Affecting Cardiomyocyte Function in Heart Failure, 35
Redox Homeostasis in the Heart, 35
Protein Synthesis, Turnover, Quality Control, and Stress Responses, 36
Endoplasmic Reticulum and the Unfolded Protein Response, 36
Autophagy, 38
Ubiquitin-Proteasome System, 38
Coordinated Functions of the Unfolded Protein Response, Ubiquitin-Proteasome System, and Autophagy Systems, 38
Cardiomyocyte Interactions with Other Cell Types, 38
Paracrine Effects of Endothelial Cells, 38
Paracrine Effects of Fibroblasts, 39
Cardiomyocyte Angiogenic Signaling, 39
Activation of Inflammatory Pathways, 39
Microrna and Long Noncoding RNA-Dependent Pathways, 41
Conclusions and Future Directions, 42
Acknowledgments
The authors’ work is supported by the British Heart Foundation; a Fondation Leducq Transatlantic Network of Excellence award; and the Department of Health via a National Institute for Health Research (NIHR) Biomedical Research Centre award to Guy’s & St Thomas’ NHS Foundation Trust in partnership with King’s College London and King’s College Hospital NHS Foundation Trust.
The development of heart failure in the context of chronic disease stresses such as hypertension or myocardial infarction (MI) is characterized initially by complex changes in the structure and function of the heart at the molecular ( see also Chapter 1 ), cellular, and organ levels. This dynamic process, termed cardiac remodeling ( see also Chapter 12 ), leads to contractile dysfunction, chamber dilatation, ventricular dyssynchrony, and arrhythmias. At the cellular level, the remodeling heart manifests significant alterations both in the cardiac myocytes and in nonmyocyte cells, such as fibroblasts, endothelial cells, and immune cells. In addition, there are significant changes in the myocardial vasculature and the composition of the extracellular matrix.
Progressive changes in the cardiac myocyte phenotype are a central abnormality in the chronically stressed and failing heart. The phenotype comprises multiple components including cell hypertrophy ( see Chapter 1 ) and alterations in calcium handling, sarcomeric function, electrical properties, redox homeostasis, metabolism, energetics, and cell viability that collectively make a major contribution to the global cardiac phenotype. Cardiomyocyte hypertrophy is also observed in physiologic settings (e.g., pregnancy or in athletes) but in this case is not accompanied by detrimental changes such as contractile impairment. This divergence in phenotypes indicates that different components of the cardiomyocyte phenotype are capable of being regulated independently, at least to some extent. Even in a disease setting (e.g., early during pressure overload), the heart may hypertrophy but maintain contractile function ( adaptive remodeling), whereas with more chronic disease stress it begins to fail. Thus the overall phenotype may be determined by the balance between potentially adaptive and maladaptive processes that occur within the cardiac myocyte.
In this chapter, we review the main cardiomyocyte cellular alterations that contribute to the pathogenesis of heart failure. We start by discussing the cellular basis for contractile dysfunction (see also Chapter 10 ) , the key cardiac manifestation of heart failure. The contribution of changes in excitation-contraction coupling (particularly calcium handling and sarcomeric function) to contractile dysfunction and arrhythmogenesis is considered. We next discuss several global alterations within cardiomyocytes that also impact on contractile function and cell viability, such as changes in redox homeostasis and signaling, cellular stress responses, and macromolecular and protein turnover. As will become evident, these global processes interact with each other and have complex effects on the remodeling process (e.g., redox homeostasis and signaling modulate excitation-contraction coupling, as well as stress responses). The cardiomyocyte phenotype in the failing heart may also be affected by other cardiac cell types and, in turn, influence those cell types. The role of cardiomyocyte interactions with other cell types—in particular fibroblasts, endothelial cells, and immune/inflammatory cells—is therefore discussed. Finally, we review the role of regulation by noncoding mRNAs such as microRNAs (miRNAs), which often have global effects on cellular signaling pathways in the failing heart. The signaling pathways that underlie the development of myocyte hypertrophy per se are discussed elsewhere in this edition. Likewise, the important role of alterations in myocardial energetics and metabolism, which, for example, may have a major impact on contractile function during heart failure, is covered in other chapters.
Contractile Dysfunction
Cardiac myocytes in the failing heart exhibit several abnormalities of contractile function, including a reduction in contractile amplitude and force of contraction, a slowing of contraction and relaxation, an increase in diastolic force, and altered responses to changes in heart rate and β-adrenergic stimulation. Perturbations in both excitation-contraction coupling and sarcomere properties contribute to these abnormalities. We provide a brief overview of normal excitation-contraction coupling and sarcomeric function and then address the distinct abnormalities that occur in heart failure.
Normal Excitation-Contraction Coupling
Physiologic cardiac function requires the coordinated temporal and spatial activation of the heart. At a cellular level, this finely tuned process is regulated mainly by accurately synchronized Ca 2+ fluxes in every cardiomyocyte ( Fig. 2.1 ). When an action potential depolarizes the cell, voltage-dependent L-type Ca 2+ channels (LTCCs) located mainly in the transverse tubules (T-tubules) open to generate an inward Ca 2+ current (I Ca ), which induces a localized increase of Ca 2+ in the “dyadic cleft” in close neighborhood to the Ca 2+ release or ryanodine receptor channels (RyR2) of the sarcoplasmic reticulum (SR). This transsarcolemmal Ca 2+ influx activates the RyR2 and results in so-called Ca 2+ -induced Ca 2+ release from the SR, which provides the major component of the increase in cytosolic Ca 2+ during systole (i.e., the “Ca 2+ transient”). Intracellular Ca 2+ concentration increases from approximately 100 nmol/L during diastole to approximately 1 μmol/L during systole and causes myofilament activation and contraction. Repolarization of the membrane potential is induced by inactivation of I Ca and the activation of delayed rectifying K + currents. During diastole, Ca 2+ is removed from the cytosol via two major pathways: (1) the SR Ca 2+ ATPase (SERCA2a) located in the membrane of the SR, which pumps Ca 2+ back into the SR lumen; (2) the sarcolemmal Na + /Ca 2+ exchanger (NCX1), which transfers Ca 2+ into the extracellular space. Through these two Ca 2+ transport mechanisms, [Ca 2+ ] i decreases to physiologic resting concentrations of approximately 100 nmol/L, allowing the cell to relax and to regain its physiologic diastolic resting cell length.

Impaired Ca 2+ Handling in Failing Cardiac Myocytes
Prolongation of the action potential duration, depressed force generating capacity, and slowed contraction and relaxation rates are the hallmark functional changes of the failing human heart. Impaired Ca 2+ handling is a key feature of the failing cardiac myocyte, with great pathophysiologic relevance for the progressive deterioration in contractile function of the failing heart. Distinct alterations in the expression levels, as well as posttranslational modifications of important cardiac Ca 2+ -handling proteins, causatively contribute to systolic and diastolic contractile dysfunction and to an increased propensity for cardiac arrhythmias. The posttranslational modifications that alter the function of key Ca 2+ -handling proteins include alterations in phosphorylation, nitrosylation, oxidation status, and sumoylation. Altered protein phosphorylation occurs secondary to changes in the activity of various kinases (e.g., cAMP-dependent protein kinase [PKA], calcium-calmodulin-dependent kinase II [CaMKII]), as well as perturbations in phosphatase (e.g., protein phosphatase 1 [PP1]) activity.
The failing cardiac myocyte has a significantly diminished amplitude of the systolic Ca 2+ transient as compared with nonfailing control myocytes ( Fig. 2.2 ), which is a major factor responsible for the reduced contractile amplitude of the failing cell ( systolic dysfunction, see Chapter 10 ). Failing myocytes typically also exhibit a slowed decay of the Ca 2+ transient during diastole, which is a major contributor to abnormal (delayed) relaxation. In addition, the normal increase in amplitude of Ca 2+ transient (and therefore force of contraction) that occurs with faster heart rate is blunted or even reversed in the failing heart (i.e., the normal positive force-frequency relationship [FFR] is converted to a flat or a negative FFR). It is generally accepted that a reduction in the Ca 2+ content of the SR is a major reason for the diminished amplitude of the systolic Ca 2+ transient and the abnormal FFR. A decreased SR Ca 2+ content has been consistently observed in myocytes isolated from failing human and animal hearts, whereas alterations in LTTC-mediated Ca 2+ influx appear to be less relevant. From a mechanistic point of view, a reduction in SR Ca 2+ content can result either from insufficient diastolic Ca 2+ refilling (or loading) of the SR or from an increased loss of Ca 2+ via the RyR2 Ca 2+ release channels during diastole and may also be influenced by changes in NCX activity. In fact, all three mechanisms may contribute to the reduction in SR Ca 2+ content and contractile phenotype of the failing myocyte ( Fig. 2.3 ).


Reduced SR Ca 2+ Reuptake in Heart Failure (see also Chapter 1 )
During diastole, SERCA2a pumps Ca 2+ into the SR lumen and provides a sufficient Ca 2+ content to be released during the subsequent systolic heartbeat. SERCA2a-dependent diastolic Ca 2+ uptake into the SR normally dominates over transsarcolemmal Ca 2+ extrusion via the NCX. SERCA2a is subject to regulation by the phosphoprotein phospholamban (PLB), which on phosphorylation by CaMKII (at Thr-17) and/or PKA (at Ser-16) releases its inhibitory effect on SERCA2a because of a dissociation of the PLB/SERCA2a complex. SERCA2a protein levels are reduced in failing myocardium, which is paralleled by a reduction in SERCA2a sumoylation, and results in an impairment of diastolic Ca 2+ reuptake into the SR. Moreover, the levels of PLB are unaltered and its phosphorylation state may be reduced, so that there is greater relative inhibition of SERCA2a by nonphosphorylated PLB, thereby aggravating the impairment of Ca 2+ reuptake ( see Fig. 2.3 ). The decrease in SR Ca 2+ content results in less Ca 2+ available for the subsequent systolic Ca 2+ transient and impairs systolic function.
Decreased SERCA2a expression may also affect diastolic function of the failing heart. If no other mechanism (such as an increased NCX activity [see later discussion]) compensates for the reduction in SERCA2a function with respect to removal of Ca 2+ from the cytosol during diastole, then there is diastolic cytosolic Ca 2+ overload. Myocytes that have increased diastolic Ca 2+ levels will have persistent low-level myofilament activation at a time when the myofilaments should be fully relaxed, resulting in increased diastolic force and diastolic dysfunction. This failure to relax fully during diastole impairs the filling of the heart and thereby may also worsen systolic dysfunction. Moreover, the abnormally elevated diastolic Ca 2+ levels may have multiple other effects, such as changes in gene transcription, cell viability, and mitochondrial function through altered activation of Ca 2+ -dependent kinases (e.g., CaMKII), phosphatases (e.g., calcineurin), mitochondrial enzymes, caspases, and other mechanisms.
In view of these effects on contractile function, as well as other aspects of the failing heart phenotype, restoring SERCA2a function in heart failure might represent a promising therapeutic approach. Experimental studies showed that adenoviral overexpression of SERCA2a in human cardiomyocytes can improve cardiac contractility because it restores SR Ca 2+ content and the systolic Ca 2+ transient, whereas reduced cytosolic Ca 2+ levels preserve diastolic function. In addition, SERCA2a overexpression was shown to improve myocardial energetics and endothelial function and to have antiarrhythmic effects. The potential clinical relevance of SERCA2a stimulation has been addressed in the CUPID trials (Calcium Up-Regulation by Percutaneous Administration of Gene Therapy in Cardiac Disease) in which patients with heart failure were treated with a single infusion of an adeno-associated viral (AAV) vector delivering SERCA2a versus placebo. Although this initial study suggested potential efficacy, the larger study CUPID2 trial failed to show efficacy in advanced heart failure.
Increased NCX Activity in the Failing Heart
The NCX is localized at the cardiomyocyte sarcolemma where, in its “forward mode,” it transfers one Ca 2+ ion into the extracellular space in exchange for three Na + ions using the transmembrane gradient for Na + ( see Fig. 2.1 ). This mechanism is electrogenic because it results in the net movement of one positive charge into the cytosol and can therefore depolarize the membrane potential and even have arrhythmogenic effects under conditions of spontaneous and localized rises in [Ca 2+ ] i (see later discussion). NCX expression and activity are found to be increased in human and experimental heart failure, which may have complex functional effects depending on the mode of NCX activity and stage of heart failure. In the face of downregulated SERCA2a function, enhanced NCX activity competes with SERCA2a for Ca 2+ elimination during diastole. This may further aggravate the decrease in SR Ca 2+ content because less cytosolic Ca 2+ is available for SERCA2a-mediated SR Ca 2+ loading. However, the increase in NCX function can also partly protect cardiac myocytes against severe diastolic Ca 2+ overload and diastolic dysfunction. Indeed, an increase in NCX levels in explanted human myocardial samples was found to correlate with a preservation of diastolic function, whereas patients with diastolic dysfunction had decreased NCX levels. On the other hand, NCX activity can contribute to Ca 2+ overload in settings where there is intracellular Na + overload. The reason is that at high [Na + ] i , NCX switches to a “reverse mode” and pumps out Na + in exchange for Ca 2+ . The increased contribution of NCX-dependent Ca 2+ influx, as opposed to SR Ca 2+ release during systole in failing myocytes, has adverse effects on mitochondrial Ca 2+ uptake (which relies on high Ca 2+ gradients), and promotes increased mitochondrial reactive oxygen species (ROS) levels because of reduced activity of Ca 2+ -dependent Krebs cycle dehydrogenases that normally maintain antioxidant reserves. This detrimental mechanism can become further aggravated in a ROS-dependent manner and lead to a vicious cycle of impaired cytosolic and mitochondrial Ca 2+ fluxes and increased oxidative stress because ROS induce further cytosolic Na + overload.
“Leaky” RyR2 Cause Diastolic SR Ca 2+ Loss in Heart Failure
Diastolic “leak” of Ca 2+ from the SR due to a pathologic increase in RyR2 open probability is an important mechanism that contributes to the lowering of SR Ca 2+ content in heart failure. Ca 2+ leaks from the SR through spontaneous and uncoordinated Ca 2+ release events or “Ca 2+ sparks.” The expression of RyR2 itself appears to be unchanged in heart failure, but its functional regulation is dramatically altered by complex posttranslational modifications. These alterations involve an increase in RyR2 phosphorylation as a result of hyperactive protein kinases, such as CaMKII and PKA, and possibly reduced RyR2 dephosphorylation. An increase in RyR2 oxidation or nitrosylation as a consequence of increased oxidative and nitrosative stress in heart failure may also be important. Although transient phosphorylation- and redox-dependent regulation of RyR2 gating may fulfill physiologic functions in healthy myocytes, in the failing myocyte the hyperphosphorylation and/or oxidation of RyR2 leads to severe diastolic SR Ca 2+ leakage. Furthermore, the coupling of LTCC to RyR is also impaired in heart failure because of T-tubule remodeling, such that some RyR are “orphaned” and contribute to dyssynchronous Ca 2+ release.
The precise mechanisms of RyR2 hyperphosphorylation and the kinases responsible for this abnormality are important to establish because they may represent therapeutic targets but are still a matter of debate. Although one laboratory reported strong evidence for a PKA-mediated dysregulation of the RyR2 in heart failure, others failed to show an increase in PKA-dependent hyperphosphorylation. There is also evidence for an involvement of CaMKII-dependent RyR2 phosphorylation in inducing SR Ca 2+ leak. Redox-related dysregulation of RyR2 opening in the failing heart may involve increased ROS produced by mitochondria or other sources such as nicotinamide adenine dinucleotide phosphate (NADPH) oxidases ( see also Chapter 8 ), and is related to the oxidation of specific cysteine residues within the RyR2. Interestingly, both the protein kinases implicated in RyR2 hyperphosphorylation (i.e., CaMKII and PKA) are subject to redox activation, so that alterations in the redox milieu of failing myocytes may also exert indirect effects on RyR2. In this regard, it is interesting to note that increased ROS in heart failure also impact adversely on SERCA2a function and other aspects of Ca 2+ handling in the failing cell ( see Fig. 2.2 ). More recently it has been shown that an increase in calcium leak from RyR2 results in mitochondrial calcium overload, which in turn has been linked to heart failure progression.
Contribution of Impaired Ca 2+ Handling to Arrhythmia
Impaired cardiomyocyte Ca 2+ handling not only leads to systolic and diastolic dysfunction but also contributes to the development of arrhythmias in heart failure. The dysregulation of RyR2 Ca 2+ release is of particular relevance in this regard. The enhanced diastolic SR Ca 2+ leak in failing cells and the accompanying spatially and temporally uncoordinated increases in intracellular Ca 2+ may drive the NCX to exchange Ca 2+ for Na + , thereby inducing an influx of positive charge (I ti ) that partly depolarizes the cell during phase 4 of the action potential ( see Fig. 2.3 ). These spontaneous depolarizations of the membrane potential are termed delayed afterdepolarizations (DADs) and are arrhythmogenic. Because NCX expression and activity are increased in heart failure, the depolarizing influx of positive charge (I ti ) will be greater for any given spontaneous Ca 2+ release from the SR. Thus the combination of leaky RyR2 and increased NCX activity synergizes to enhance ventricular arrhythmogenesis. Similar mechanisms may also contribute to the development of atrial fibrillation, the occurrence of which is higher in heart failure. There is a significant potential for feedback among the different ionic mechanisms that contribute to arrhythmogenesis in the failing cardiomyocyte and therefore the possibility of a self-sustaining vicious cycle of hampered Ca 2+ and Na + handling that contributes to contractile dysfunction and arrhythmia. For example, if the NCX starts to function in reverse mode because of elevated intracellular [Na + ], the resulting increase in Ca 2+ influx may activate proarrhythmogenic kinases such as CaMKII and lead to further arrhythmogenic SR Ca 2+ leakage through phosphorylation of RyR2.
Sarcomeric Dysfunction in Heart Failure
Cardiac mechanical activity occurs as a result of the interaction between changes in cytosolic Ca 2+ concentration and the contractile myofilaments ( Fig. 2.4 ). Contractile function is influenced not only by changes in Ca 2+ concentration but also by the intrinsic myofilament responsiveness to Ca 2+ , which is dependent on the properties of the actomyosin complex and regulatory proteins such as the troponin complex and myosin-binding protein C. During diastole, the mechanical interaction between actin and myosin is inhibited by the tropomyosin-troponin complex. When cytosolic Ca 2+ concentration increases during systole, the binding of Ca 2+ to troponin C (cTnC) relieves this inhibitory effect and allows actomyosin interaction and contraction to occur. A subsequent decrease in cytosolic Ca 2+ leads to its release from cTnC and muscle relaxation. Other components of the sarcomere also affect the mechanical properties of heart muscle. In particular, the giant filamentous protein titin—which connects the Z disc to the M band and confers significant “elasticity” to the sarcomere—has an important influence on passive muscle stiffness, which in turn is an important determinant of diastolic function.

Significant evidence suggests that alterations in myofilament properties contribute to systolic and diastolic contractile dysfunction in heart failure. Perhaps the best-known contribution of myofilament properties to contractile changes in the stressed and failing heart is the shift of myosin heavy chain (MHC) from the fast α-MHC to a slower β-MHC isoform. The significantly lower ATPase activity of β-MHC is beneficial in that it is more energetically efficient but at the same time may result in slower relaxation and lower contractility. This isoform shift is a prominent feature in rodent models, where α-MHC is the dominant isoform in healthy myocardium, but may be less important in human heart failure because β-MHC dominates over α-MHC in healthy human ventricular myocardium. Nevertheless, a small impact of such an MHC isoform shift is suggested in failing human ventricular tissue.
A major regulator of myofilament Ca 2+ sensitivity in the normal heart is the PKA-mediated phosphorylation of troponin I (cTnI), which results in a lower affinity for Ca 2+ and contributes to faster kinetics of myofilament cross-bridge cycling (i.e., faster contraction and relaxation of myocytes). In the failing heart, PKA-dependent phosphorylation of cTnI is generally decreased (related to reduced β-adrenergic responsiveness) and results in increased myofilament Ca 2+ sensitivity. The major effect of such an increase in myofilament Ca 2+ sensitivity is thought to be to impair relaxation and aggravate the slowed kinetics of Ca 2+ transient decay in the failing myocyte ( see Fig. 2.4 ). Similar functional effects on myofilament Ca 2+ sensitivity have been reported when there is C-terminal truncation of cTnI after myocardial ischemia/reperfusion injury, and these could play a particular role in ischemic heart failure. The phosphorylation of myosin-binding protein C is thought to play a role in the physiologic enhancement of rate of contraction and relaxation observed after β-adrenergic stimulation. Therefore hypophosphorylation of myosin-binding protein C may also contribute to contractile dysfunction.
Another shift in protein isoform that is reported in failing hearts is a shift from the stiffer (i.e., less elastic) N2B titin isoform to a more compliant N2BA isoform. It is suggested that this shift may counterbalance the decreased phosphorylation status of titin in the failing human heart, which functionally results in an increased passive stiffness. The underlying defect in titin phosphorylation is thought to be PKA dependent (as with reduced cTnI and myosin-binding protein C phosphorylation), but dysfunctional CaMKII-dependent phosphorylation of titin is also reported. Cyclic GMP-dependent protein kinase (PKG), activated by nitric oxide (NO), may also phosphorylate cTnI and titin and have similar effects to PKA. In heart failure, there is usually a reduction in NO/PKG activity, which promotes increased titin-dependent passive stiffness. Finally, changes in the redox milieu within the failing myocytes may also contribute to contractile dysfunction (e.g., through specific oxidative modifications in titin that lead to increased passive stiffness).
Global Mechanisms Affecting Cardiomyocyte Function in Heart Failure
Redox Homeostasis in the Heart (see also Chapter 8 )
ROS are generated in cardiac myocytes (as in other cell types) either as a by-product of cellular respiration and metabolism or through specialized enzymes. An important physiologic function of ROS is in redox signaling (i.e., the highly specific, usually reversible oxidation/reduction modification of signaling molecules involved in various homeostatic processes). Redox signaling is tightly regulated in a spatially and temporally confined manner and depends on appropriate inactivation or scavenging of ROS by cellular antioxidants to terminate the ROS signal. Another critical function of ROS is their involvement in oxidative protein folding in the endoplasmic reticulum (ER). In pathologic settings such as heart failure, physiologic redox signaling pathways may be perturbed or different redox-sensitive signaling pathways may be activated. Altered redox homeostasis in the ER may have a major impact on protein synthesis and stress responses (see later). In addition, an imbalance between ROS production and antioxidant reserves (i.e., oxidative stress) can result in nonspecific detrimental effects because of the irreversible oxidation of macromolecules, membranes, and DNA. Therefore alterations in redox homeostasis in the failing cardiomyocyte have a profound impact on many aspects of the myocyte phenotype.
Major sources of ROS in failing cardiac myocytes include the mitochondrial electron transport chain (ETC), NADPH oxidase proteins (NOXs), monoamine oxidases, uncoupled NO synthases (NOSs), and xanthine oxidases. Pathophysiologically important ROS species include superoxide, hydrogen peroxide (produced by dismutation of superoxide), hydroxyl ions, and peroxynitrite generated by the reaction of superoxide with NO. Electron leak from the mitochondrial ETC generates superoxide, and this becomes quantitatively more important in heart failure as a result of ETC dysfunction, uncoupling, and an impairment of mitochondrial antioxidants. Mitochondrial ROS generation can lead to opening of the mitochondrial permeability transition pore (MPTP) and loss of cell viability. Furthermore, such ROS production may also induce further mitochondrial ROS production, termed ROS-induced ROS release . Monoamine oxidases, which catabolize the neurotransmitters noradrenaline and serotonin, have recently been recognized as additional important mitochondrial sources of ROS in the failing heart. Nonmitochondrial ROS sources, such as NOXs and xanthine oxidase, may also stimulate mitochondrial ROS production. Xanthine oxidase–derived ROS may be particularly important in the setting of ischemia-reperfusion. The inhibition of mitochondrial ROS using various mitochondrially targeted agents is considered to be a promising therapeutic approach in heart failure.
NOX proteins are especially important for redox signaling, being the only source that has a primary ROS-generating function. They catalyze electron transfer from NADPH to molecular O 2 , thereby generating superoxide and/or hydrogen peroxide. Among seven distinct NOX family members, NOX2 and NOX4 are expressed in cardiomyocytes and other cardiac cells (e.g., endothelial cells, fibroblasts, inflammatory cells). Although both isoforms generate ROS, there are significant differences in their structure, activation, and subcellular localization that contribute to important differences in function. NOX2 has been shown to have a physiologic function in stretch-induced excitation-contraction coupling, whereas NOX4 may regulate cardiomyocyte differentiation. The activities of both NOX2 and NOX4 are increased in heart failure, the former mainly as a result of increased activation by stimuli, such as angiotensin II and cytokines, and the latter largely because of increased expression levels. An additional Ca 2+ -sensitive isoform, NOX5, may be important in the human heart.
NOS enzymes normally catalyze the production of NO from L -arginine. Cardiomyocytes constitutively express neuronal (nNOS) and endothelial (eNOS) isoforms, which have distinct physiologic actions, notably in regulating excitation-contraction coupling and inotropic responsiveness. An inducible iNOS isoform is upregulated in response to cytokine stimulation. During heart failure, NOSs can become “uncoupled” and switch from NO to superoxide generation, resulting in loss of the normal NO-mediated effects, as well as detrimental effects related to ROS production. Partial uncoupling results in the simultaneous generation of NO and superoxide, leading to peroxynitrite production. Uncoupling of NOSs is usually related to a reduction in the availability of the cofactor tetrahydrobiopterin. In the case of eNOS, there additionally is S -glutathionylation of specific cysteine residues in the reductase domain. Importantly, both these mechanisms are enhanced by oxidative stress so that NOS uncoupling can often act to amplify ROS generation by other sources.
Alterations in antioxidant balance are an important contributor to altered redox homeostasis in the failing heart. These include changes in antioxidant enzymes, such as superoxide dismutases, catalase, glutathione peroxidase, thioredoxin, peroxiredoxin, glutathione S -transferases, and others. A crucial factor for redox homeostasis is the level of NADPH in different cellular compartments, with this nucleotide being required for the regeneration of reduced pools of major cellular antioxidants, such as glutathione, glutaredoxin, and thioredoxin. Therefore metabolic reactions that generate NADPH have a potentially broad impact on the myocyte phenotype. In the mitochondria, the activity of Ca 2+ -dependent dehydrogenases is important in this regard, and this is inhibited by cytosolic Na + overload in heart failure as mentioned earlier. In the cytosol, glucose-6-phosphate dehydrogenase (G6PD) is a key rate-limiting enzyme involved in NADPH production and has been shown to affect cardiomyocyte calcium homeostasis and contractile dysfunction in ischemia-reperfusion. Interestingly, excessively high levels of NADPH and glutathione can be detrimental by inducing so-called reductive stress. In a mouse model of mutant αB-crystallin (CryAB) cardiomyopathy, such reductive stress is associated with abnormal protein folding and the accumulation of protein aggregates in cardiomyocytes.
Altered redox homeostasis affects an extensive range of molecular targets in the failing cardiomyocyte, including kinases, phosphatases, ion transporters and channels, myofilaments, and transcription factors, as reviewed in detail elsewhere. Here we discuss a few examples where the mechanisms of such redox modifications and their impact on the myocyte phenotype have been addressed in sufficient depth to offer the promise of therapeutically tractable targets. Redox dysregulation affects several proteins involved in abnormal excitation-contraction coupling, as discussed earlier. NOX2 appears to be an important ROS source in this regard and may act both through direct oxidation of proteins critical in excitation-contraction coupling such as RyR2 and via the modulation of redox-sensitive kinases or phosphatases that regulate such proteins. NOX2 is also implicated in the genesis of atrial fibrillation. Several signaling pathways involved in cardiomyocyte hypertrophy are in part redox-regulated (e.g., the activation of ASK-1, ERK1/2, NF-κB, and Akt). NOX2 is again an important ROS source responsible for such regulation, especially in the setting of increased renin-angiotensin system activation. Class II histone deacetylases (HDACs) are key regulators of hypertrophic gene expression, allowing this to occur when they are exported from the cardiomyocyte nucleus. A thioredoxin 1–sensitive pathway for the specific oxidation and subsequent nuclear export of HDAC4 is reported to be important in adrenergic-mediated cardiomyocyte hypertrophy. Cardiomyocyte viability may be adversely affected by an altered redox balance (e.g., leading to increased mitochondrial ROS production). In addition, the specific oxidative activation of kinases such as CaMKII has been found to be important. PKG is also oxidatively activated and was shown to be physiologically important in stretch-induced tuning of the Frank-Starling response via the phosphorylation of phospholamban, but persistent oxidation during chronic pressure overload was found to be detrimental. Not all ROS effects are detrimental in the stressed cardiomyocyte. For example, an increase in NOX4 has been shown to be cardioprotective during chronic pressure overload or ischemic stress by enhancing beneficial signaling mechanisms including myocyte hypoxia-inducible factor 1-alpha (HIF1α) signaling leading to paracrine effects on myocardial capillary density, enhancement of the activating transcription factor 4 (ATF4)-mediated integrated stress response and alterations in myocardial substrate use. This underscores the concept that the effects of altered redox homeostasis may vary depending on the ROS source and its subcellular location.
Protein Synthesis, Turnover, Quality Control, and Stress Responses
The dynamic cellular remodeling that occurs in the stressed and failing heart involves substantial changes in protein synthesis and turnover and requires robust quality control mechanisms to ensure the degradation or clearance of abnormal proteins (e.g., misfolded proteins and protein aggregates). Several organelles and systems are important for these functions, including the ER, the ubiquitin-proteasome system (UPS), and autophagy. The cell can mount specific stress responses designed to facilitate the maintenance of some of these functions (e.g., the unfolded protein response [UPR] or an enhancement of autophagy). These systems make a major contribution to the cellular phenotype in the failing heart and may represent targets for therapeutic modulation. We next discuss in more detail the role of some of these systems in the failing heart.
Endoplasmic Reticulum and the Unfolded Protein Response
The cardiac myocyte SR is a specialized ER that is especially important for intracellular Ca 2+ regulation, and this is known to become dysfunctional in heart failure, as discussed earlier in this chapter. In addition to Ca 2+ homeostasis, the ER coordinates other important functions such as the correct folding, posttranslational modification, and quality control of membrane and secreted proteins, gluconeogenesis, lipid, and steroid synthesis. It also interacts with and influences mitochondrial function (e.g., through transfer of Ca 2+ from the ER). These functions are important in cardiac myocytes as in other cell types, although the distinction and/or overlap between the SR and ER remain poorly defined. The requirement for protein folding, quality control, and related functions in the ER changes significantly in the remodeling heart where there are significant alterations in protein turnover (e.g., due to hypertrophy), metabolism, and energetics. The maintenance of appropriate ER function therefore becomes an important factor in cellular homeostasis.
The ER stress response or UPR is a conserved mechanism that senses the status of ER protein folding by detecting the accumulation of misfolded proteins and inducing a program of changes that affects both the ER and other cellular organelles and processes. The UPR encompasses homeostatic mechanisms that include the increased synthesis of chaperones, which assist the refolding of misfolded proteins, inhibition of further protein translation that may aggravate misfolding and ER overload, and the activation of degradation pathways to break down misfolded proteins. Many different stressors can induce the UPR, including glucose or amino acid deprivation, oxidative stress, hypoxia, and ionic imbalance. Induction of the UPR is mediated through three transmembrane ER stress sensors, namely protein kinase RNA-like ER kinase (PERK), inositol-requiring kinase 1 (IRE1), and activating transcription factor 6 (ATF6), that “sense” luminal ER stress and respond by activating specific transcriptional pathways while inhibiting global protein translation ( Fig. 2.5 ). Such activation of the UPR has the potential to be an adaptive response that restores homeostasis or a detrimental response that triggers cell death pathways, depending on its strength, duration, and context.
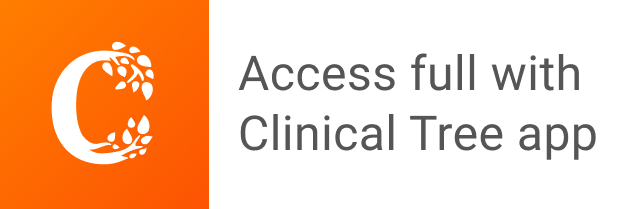