Cellular and Molecular Biology of the Lung
Steven J. Mentzer
The primary function of the lung is the gas exchange necessary to maintain aerobic metabolism. To sustain an active human adult, oxygen must be adsorbed and carbon dioxide removed. This process requires a mechanism for the ventilation of large volumes of respiratory gases. The upper airways function to conduct gas into and out of the lung. When the respiratory gases reach the alveoli, a large alveolar surface area facilitates the efficient exchange of oxygen and carbon dioxide.
The ventilation of respiratory gases results in the exposure of the lung to a variety of airborne pathogens. A complex defense system uses mechanical and immunologic mechanisms to protect the host from biological pathogens. The importance of mechanical mechanisms of mucociliary clearance is illustrated by the heritable disease cystic fibrosis. The abnormal mucus in cystic fibrosis leads to lung hyperinflation and recurrent infections. In addition to biological threats, the ventilation of gases means that the lung is also exposed to a variety of environmental toxins. Cigarette smoke is a toxin that has effects on both gas exchange (emphysema) and the cells that line the airways (bronchial carcinoma). Similar environmental exposures are responsible for other acquired diseases of the lung.
Large Conducting Airways
A variety of cells and their products ensure optimal gas exchange and limit the impact of airborne pathogens on lung function. The tracheobronchial tree is characterized by airway epithelium specialized for conductance and mucociliary clearance. A mixed population of epithelial cells lines the trachea and bronchi (Table 4-1). These epithelial cells include basal cells, goblet cells, and ciliated columnar epithelium. Characteristic of the proximal airways is the presence of ciliated columnar epithelial cells and goblet cells. Basal cells and so-called intermediate cells are also present. The function of basal cells is unclear, but these cells may give rise to both goblet cells and ciliated epithelial cells. Specialized lymphoid tissues, often referred to as bronchus-associated lymphoid tissues (BALT), are located along the main airways and especially at the bifurcation of airways where particles and pathogen deposition is concentrated.
Mucous and Serous Glands
Mucous and serous glands are present in the large airways down to the bronchiolar level. These glands are located between the muscle and the cartilage layers of the large airways. The glands are composed of both serous and mucous tubules. Although there may be some mixture, separate areas of the gland are composed of either serous or mucous tubules. These tubules end in collecting and ciliated ducts.
The mucous cells are primarily restricted to mucous tubules. The myoepithelial cells that line part of the mucous glands are responsible for expelling mucous contents into the airway lumen. The mucous glands are supplied by the sympathetic nervous system. Serous cells can be found in serous tubules and have been identified to the level of the bronchioles. The main function of serous cells is to produce lysozyme and possibly aid in the transport of immunoglobulin A (IgA) across the glandular epithelium. IgA is produced by plasma cells, which are found in the region of bronchial glands.
Goblet Cells
The goblet cell is a surface mucus-secreting cell present throughout the bronchial airways. Goblet cells produce mucus. Respiratory airway mucus is composed of glycoproteins with unique viscoelastic properties. These glycoproteins are collectively called mucins. Mucins are heterogeneous macromolecules that have domains for the passive clearance of both proteins and lipids. Mucins may also actively bind microorganisms. The mucin glycoproteins may function as ligands for lectin-like surface receptors expressed by microorganisms. The role of the goblet cell in mucin production is particularly apparent after airway injury. Exposure to cigarette smoke, for example, leads to goblet cell metaplasia as well as glandular hypertrophy. Goblet cell metaplasia may also be observed in a variety of other inhalational injuries. Mucins are overexpressed in lung diseases such as asthma, bronchitis, chronic obstructive pulmonary disease, and cystic fibrosis.
Ciliated Columnar Cells
The percentage of ciliated columnar epithelial cells is generally higher in the large airways than in the peripheral airways. About
50% of the cells in the trachea are ciliated epithelium, whereas only 15% of cells in the fifth-generation airways are ciliated. In humans, the ratio of ciliated cells to goblet cells is about 5:1. Ciliated cells propel mucus through the airways.
50% of the cells in the trachea are ciliated epithelium, whereas only 15% of cells in the fifth-generation airways are ciliated. In humans, the ratio of ciliated cells to goblet cells is about 5:1. Ciliated cells propel mucus through the airways.
Table 4-1 Epithelial Cells | |||||||||||||||||||||
---|---|---|---|---|---|---|---|---|---|---|---|---|---|---|---|---|---|---|---|---|---|
|
About 250 cilia are located on the luminal surface of each ciliated cell. The cilia are composed of an array of longitudinal microtubules called an axoneme. The axoneme is arranged with a central doublet surrounded by nine outer doublets. A sliding movement of the microtubules past each other generates the movement of the cilia. The 9 + 2 arrangement of microtubules in human cilia is similar to the structure of axonemes in other plants and animals. The clinical importance of cilia is illustrated by the syndrome primary ciliary dyskinesia (PCD). PCD can involve bronchiectasis, chronic rhinosinusitis, and poorly motile spermatozoa. Kartagener’s syndrome, which includes the diagnosis of situs inversus in addition to cilia dysfunction, is a subset of PCD.
The normal airway cilia function to propel both water and mucus. When the cilia propel mucus, the tip of the cilium penetrates the mucus and claws the mucus forward. At the end of the propulsive stroke, the cilium’s tip leaves the mucus and moves backward beneath the mucus in a recovery stroke. The average beat frequency of cilia ranges from 12 to 15 beats per minute but is sensitive to both clinical and pharmacologic factors. Neurohormonal control of ciliary beat frequency appears to be regulated by an adrenergic mechanism. Ciliated epithelial cells also appear to be sensitive to environmental injury. Cigarette smoke is associated with a loss of ciliated epithelium and replacement with squamous metaplasia. Ischemic injury of the airway, as seen after lung transplantation, is also associated with the loss of ciliated epithelium and an increase in squamous metaplasia.
Effective mucociliary clearance is critical for lung defense (Table 4-2). When ciliary activity is inadequate to remove all secretions from the airway, the physical presence of the mucus initiates a neural reflexive cough. The cough generates a high shear force that dislodges the mucus and expels it from the airway. In healthy people, mucus transport in the airways does not require a cough. In contrast, when excess mucus resides in the airway, high expiratory air velocity can play an important role in the clearance of secretions.
Table 4-2 Factors Influencing Mucociliary Clearance | |||||||||||||||||||||
---|---|---|---|---|---|---|---|---|---|---|---|---|---|---|---|---|---|---|---|---|---|
|
Effective mucociliary clearance also depends on the viscoelastic properties of the mucus. In general, the viscosity and elasticity of the mucus varies inversely with the water content. When the water content of the mucus is high, the mucus is effectively cleared without a cough. When the water content of the mucus is low, the mucus is thick and tenacious. Mucus with high viscosity and elasticity can be effectively cleared by a cough. The clinical problem of high viscosity and elasticity occurs in the patient with a poor cough or impaired airflow. For example, the patient with a paretic vocal cord or chronic obstructive lung disease may not be able to generate sufficient airflow to expel mucus with high viscoelasticity. The inability to clear the airway mucus may result in decreasing airflow and subsequent mucous impaction.
Small Conducting Airways
The terminal bronchiole represents the most distal purely conducting portion of the tracheobronchial tree. Although there is some anatomic variation, bronchioles are generally distinguished from bronchi by the fact that bronchi contain cartilage in their walls, whereas bronchioles do not. Terminal bronchioles, like the proximal airways, have an epithelial lining that is specialized for conductance and mucociliary clearance.
The transitional zone between gas exchange areas and the conducting airways is termed the bronchiolar zone. Bronchioles are the most distal conducting airways proximal to the pulmonary acinus. The epithelial lining of bronchioles is largely composed of Clara cells. These nonciliated and nonsquamous epithelial cells constitute 70% to 90% of the cells throughout the transitional bronchiolar zone.
Clara Cells
Clara cells appear to have multiple metabolic functions. The ultrastructure of the Clara cell is characterized by extensive apical projections into the airway lumen and prominent endoplasmic reticulum. A variety of functional studies have established Clara cells as a primary site of xenobiotic metabolism. The Clara cell may also function as a secretory cell for the terminal airways. Clara cell secretory granules may be a source of surfactant apoproteins in the human lung. Clara cells may also serve as a source of arachidonic acid metabolites and antileukoproteases.
The turnover of epithelial cell populations in the bronchiolar region of the lung is very low. With injury, however, there is a dramatic increase in epithelial proliferative activity. Clara cells appear to function as a progenitor of themselves as well as
ciliated cells in the bronchioles. The bronchiolar ciliated cell appears to be a principal target of oxidant gases. As bronchiolar ciliated cells are injured, there is proliferation of bronchiolar Clara cells. The hyperplasia of Clara cells may effectively increase the number of respiratory bronchioles by several airway generations. The relationship of Clara cell hyperplasia to bronchiolitis and obliterative small airway processes is unknown.
ciliated cells in the bronchioles. The bronchiolar ciliated cell appears to be a principal target of oxidant gases. As bronchiolar ciliated cells are injured, there is proliferation of bronchiolar Clara cells. The hyperplasia of Clara cells may effectively increase the number of respiratory bronchioles by several airway generations. The relationship of Clara cell hyperplasia to bronchiolitis and obliterative small airway processes is unknown.
Alveolar Cells
The terminal bronchioles give rise to respiratory bronchioles. The respiratory bronchioles are not only conducting airways but also give rise to alveolar ducts that are studded with alveoli. Alveoli are the true gas-exchange surfaces of the lung. The alveoli are composed of specialized epithelial and endothelial cells separated by an interstitial matrix. Associated with these cells are alveolar macrophages. These are pivotal regulatory cells in the host defense of the distal airway.
Alveolar Type I Cells
The alveolar type I cells are the dominant component of a continuous layer of alveolar epithelium. These cells form a thin membrane over 90% of the alveolar surface. The alveolar type I cell is broad and flat, with highly branched cytoplasmic processes. Ultrastructural studies have shown that alveolar type I cells have small nuclei and very few mitochondria. This simplified cellular machinery is believed to be associated with terminal differentiation. Because of their inability to divide, alveolar type I cells are dependent on alveolar type II cells for their replacement.
Alveolar type I cells provide an important barrier to the leakage of water and solutes out of the blood and into the airspaces. This function is the result of tight junctions between alveolar cells. The alveolar tight junctions form a continuous seal between the luminal and abluminal compartments. In addition to serving a barrier function, tight junctions may regulate the polarity of the cell membrane.
Because the alveolar type I cell is incapable of mitosis and repair, it is very sensitive to injury. In most models of acute lung injury, alveolar type I cells are the first cells to be damaged. Damaged alveolar type I cells detach from the epithelium, leaving behind a denuded basement membrane. The basement membrane alone provides a poor mechanical barrier. The consequence of a loss of alveolar type I cells is edema and hemorrhage into the alveolar spaces. Subsequent impairment in gas exchange persists until the proliferation of the alveolar type II cells can replace the lost alveolar type I cell population.
Alveolar Type II Cells
Alveolar type II cells constitute about 15% of the cells in the distal lung. The cells have a distinctive appearance by light microscopy. In contrast to the squamous alveolar type I cells, alveolar type II cells are cuboidal in shape. The intracellular stores of surface-active material give the alveolar type II cells a distinctive granular appearance. This distinctive appearance has led to the description of alveolar type II cells as granular pneumocytes.
The primary function of alveolar type II cells is the synthesis and secretion of surface-active material. Alveolar type II cells contain unique organelles called lamellar bodies, which contain layers of surfactant phospholipids surrounded by a limiting membrane. Lamellar bodies also contain lysosomal enzymes and surfactant proteins. The lipid contained in the lamellar bodies is secreted at the cellular apex. The lamellar body fuses with the apical cell membrane, and the surfactant is released into alveolar space. After the release of surfactant lipids, the spheroid lamellar bodies appear to reorganize into a structure called tubular myelin, which may function to aid in adsorption and facilitate the distribution of surfactant along the alveolar surface.
Alveolar type II cells may also play an important role in the maintenance of the alveolar epithelium by their ability to differentiate into alveolar type I cells. The repair of injured alveolar epithelium occurs by the proliferation of alveolar type II cells. The proliferating type II cells appear to be capable of differentiating into either new alveolar type II cells or squamous alveolar type I cells. The differentiation of the proliferating alveolar type II cells appears to be regulated by the extracellular matrix. The connective tissue that supports the alveolar epithelium likely provides the signals that control differentiation.
Alveolar type II cells also appear to be important in disease. Alveolar type II cells are morphologically hyperplastic after lung injury. The appearance of alveolar type II cells by light microscopy has led to the term reactive pneumocytes. Reactive pneumocytes also expressed more class I and II major histocompatibility complex (MHC) images. The expression of increased levels of MHC suggests that alveolar type II cells may have an immunologic function or play a role in local autoimmune processes.
Surfactant
Surfactant plays an important role in modulating the surface forces in the alveolus. It forms a film at the surface of the alveolar lining fluid. The effect of surfactant on surface tension is particularly important at low lung volumes. In early inspiration, surfactant promotes lung expansion by reducing alveolar surface tension. At end expiration, the reduction in surface tension at low transpulmonary pressures prevents atelectasis and lung collapse.
Surfactant is composed of several heterogeneous phospho-lipid-rich lipoproteins. In the alveolus, surfactant includes the surface phospholipid monolayer, tubular myelin, as well as an apoprotein component. The dominant component of surfactant is phospholipids (Table 4-3). Phospholipids are amphipathic molecules with a polar head attached to a glyceryl
backbone. Acyl chains of variable length are attached to this backbone. In an aqueous environment, such as the alveolus, the phospholipids generally exist as a closed bilayer. Although the behavior of phospholipids can vary with composition, the general characteristic of phospholipid mixtures is that they spontaneously form a surface film at an air–fluid interface. The formation of this surface film significantly lowers surface tension. When the surface area decreases, as in expiration, the phospholipid molecules are packed more tightly, further lowering surface tension.
backbone. Acyl chains of variable length are attached to this backbone. In an aqueous environment, such as the alveolus, the phospholipids generally exist as a closed bilayer. Although the behavior of phospholipids can vary with composition, the general characteristic of phospholipid mixtures is that they spontaneously form a surface film at an air–fluid interface. The formation of this surface film significantly lowers surface tension. When the surface area decreases, as in expiration, the phospholipid molecules are packed more tightly, further lowering surface tension.
Table 4-3 Components of Surfactant | |||||||||
---|---|---|---|---|---|---|---|---|---|
|
The protein composition of surfactant appears to play an important role in surfactant function. The most abundant surfactant protein is SP-A. SP-A is a large collagen-like glycoprotein that makes up about 4% of the total mass of isolated surfactant. Surfactant is produced in the alveolar type II cells and perhaps is also synthesized in Clara cells. The chemical interaction between SP-A and surfactant lipids is complex. SP-A may play a role in regulating the secretion and turnover of surfactant. Two additional surfactant apoproteins, SP-B and SP-C, are remarkably hydrophobic. Because of their hydrophobicity, these two apoproteins are often referred to as surfactant proteolipids. Both of these proteins are thought to play a role in the formation of the surfactant film.
The functional role of surfactant system is illustrated in several pathologic conditions. The acute respiratory distress syndrome is respiratory failure secondary to atelectasis that accompanies premature birth, which is associated with a deficiency of the surfactant system. When these infants are treated with exogenous surfactant, there is a dramatic improvement in the mechanical properties of their lungs. The reduction in surface tension associated with the exogenous surfactant therapy results in pulmonary inflation and a dramatic improvement in alveolar ventilation.
Endothelial Cells
Endothelial cells in the lung form a continuous and nonfenestrated vascular lining extending from the pulmonary arteries, through an intervening capillary network, to the pulmonary veins. The blood vessels in the lung are unique vessels in the body because they are low-resistance vessels that carry deoxygenated blood on the arterial side and oxygenated blood on the venous side of the circulation. Endothelial cells compose 40% of all lung cells. The endothelial cells of the lung form a continuous sheet with an area of 130 m2 in an average adult. In the alveolar capillaries, endothelial cells have specialized organelle-free cytoplasm, only 35 to 55 nm thick, to facilitate gas exchange.
Endothelial cells generally orient in the long axis of the vessel, suggesting a morphologic response to existing shear forces. Like epithelial cells, endothelial cells have both luminal and abluminal domains to the cell membrane. These cell membrane domains are separated by intercellular tight junctions. Luminal domains have distinct functional characteristics. Proteins that regulate a variety of metabolic functions are expressed on the luminal surface (Table 4-4) and frequently associated as “rafts” in the lipid membranes. In addition, luminal domains appear to direct the secretion of cellular products, including von Willebrand’s factor. The abluminal cell membranes interact with extracellular matrix and direct transport of plasma molecules toward the interstitium.
Table 4-4 Endothelial Cell Surface Proteins and Enzymes | |
---|---|
|
The luminal membrane of endothelial cells is covered by a “fuzzy coat,” or glycocalyx, composed of glycosaminoglycans, oligosaccharide moieties of glycoproteins, glycolipids, and sialoconjugates. The cell membrane and its glycocalyx regulate a variety of cell functions. The luminal cell membrane mediates all cellular interactions and regulates recruitment of leukocytes into the lung. Enzymes such as angiotensin-converting enzyme, lipoprotein lipase, and receptors for insulin and low-density lipoproteins are expressed at the blood interface. Plasma proteins such as immunoglobulin, fibrinogen, fibrin, alpha2 macroglobulin, and albumin can be temporarily associated with the cell surface.
Alveolar Macrophages
The most common immune cell in the lung is the alveolar macrophage. This macrophage is 5 to 10 times more common in the lung than are T lymphocytes. Alveolar macrophages appear to have multiple functions in the lung. A primary function is their ability to scavenge particles and remove debris from the lung parenchyma. The ability of alveolar macrophages to phagocytize microorganisms provides an important defense against airborne pathogens. The alveolar macrophage also appears to play an important role in the repair and maintenance of lung parenchymal tissue.
Macrophages are far more common in the lower respiratory tract than in the proximal airways. Alveolar macrophages are believed to be derived from blood monocytes that migrate from pulmonary capillaries into the lung; they are thought to have a limited potential to divide and proliferate. The significant increase in alveolar macrophage concentration in some conditions, such as granulomatous lung disease, suggests either active recruitment of blood monocytes or the ability of alveolar macrophages to proliferate in situ.
Although alveolar macrophages can have several different phenotypes, subpopulations of alveolar macrophages have not been clearly defined. The reason for this discrepancy is that alveolar macrophages can exist at a variety of different activation states. The activation states of alveolar macrophages appear to regulate the capacity to phagocytize, kill target cells, migrate, and release a broad range of secretory products. The activation signals for alveolar macrophages can include such diverse signals as the phagocytosis of inert particles, receptor binding of immunoglobulin, or exposure to chemokines.
Alveolar macrophages play an important role in maintaining the sterility of the airway. Airborne bacterial pathogens can be phagocytized as inert particles or by specific surface receptors on the alveolar macrophage membrane. Surface receptors
may include membrane-bound immunoglobulin or receptors for terminal mannose sugars. Once the pathogen is phagocytized, the phagosome fuses with lysosome, and the organism is killed by an oxidative burst. Alveolar macrophages also use nonoxidative mechanisms, including proteases, lysozymes and a variety of other bacteriocidal proteins. An understanding of macrophage-dependent mechanisms of bactericidal activity holds the promise of novel antibacterial therapies.
may include membrane-bound immunoglobulin or receptors for terminal mannose sugars. Once the pathogen is phagocytized, the phagosome fuses with lysosome, and the organism is killed by an oxidative burst. Alveolar macrophages also use nonoxidative mechanisms, including proteases, lysozymes and a variety of other bacteriocidal proteins. An understanding of macrophage-dependent mechanisms of bactericidal activity holds the promise of novel antibacterial therapies.
The effectiveness of alveolar macrophages to eliminate microorganisms is varied: some microorganisms are susceptible to alveolar macrophages, whereas others are resistant. Some common bacterial pathogens, such as Staphylococcus aureus, are readily eliminated by alveolar macrophages. In contrast, Pseudomonas aeruginosa and Klebsiella pneumoniae are relatively resistant to alveolar macrophages and require the presence of neutrophils for elimination. The antimicrobial selectivity of alveolar macrophages can be of clinical importance. For example, patients who have neutropenia from chemotherapy have an enhanced susceptibility to macrophage-resistant bacteria. Other resistant infectious agents include organisms such as Mycobacterium tuberculosis and Toxoplasma gondii. These organisms can continue to grow within alveolar macrophages. Activation of alveolar macrophages, with cytokines such as interferon-gamma, can be effective in suppressing the growth of these pathogens.
Alveolar macrophages also play an important role in eliminating damaged lung tissue and airway debris. The role of the alveolar macrophage in maintaining the normal structure of the lung is illustrated by several clinical examples. Alveolar proteinosis is a disease characterized by hypoxemia from large amounts of proteinaceous material found within the alveolar air spaces. The alveolar macrophages in these patients are filled with surfactant-like material—a situation likely reflecting impairment in surfactant turnover. Another example is the anthracosis observed in long-term smokers. The anthracotic material found in bronchoalveolar lavage specimens, as well as in the histologic examination of the peripheral lung, is commonly found within alveolar macrophages. The macrophage appears to be the primary mode of elimination of airway debris.
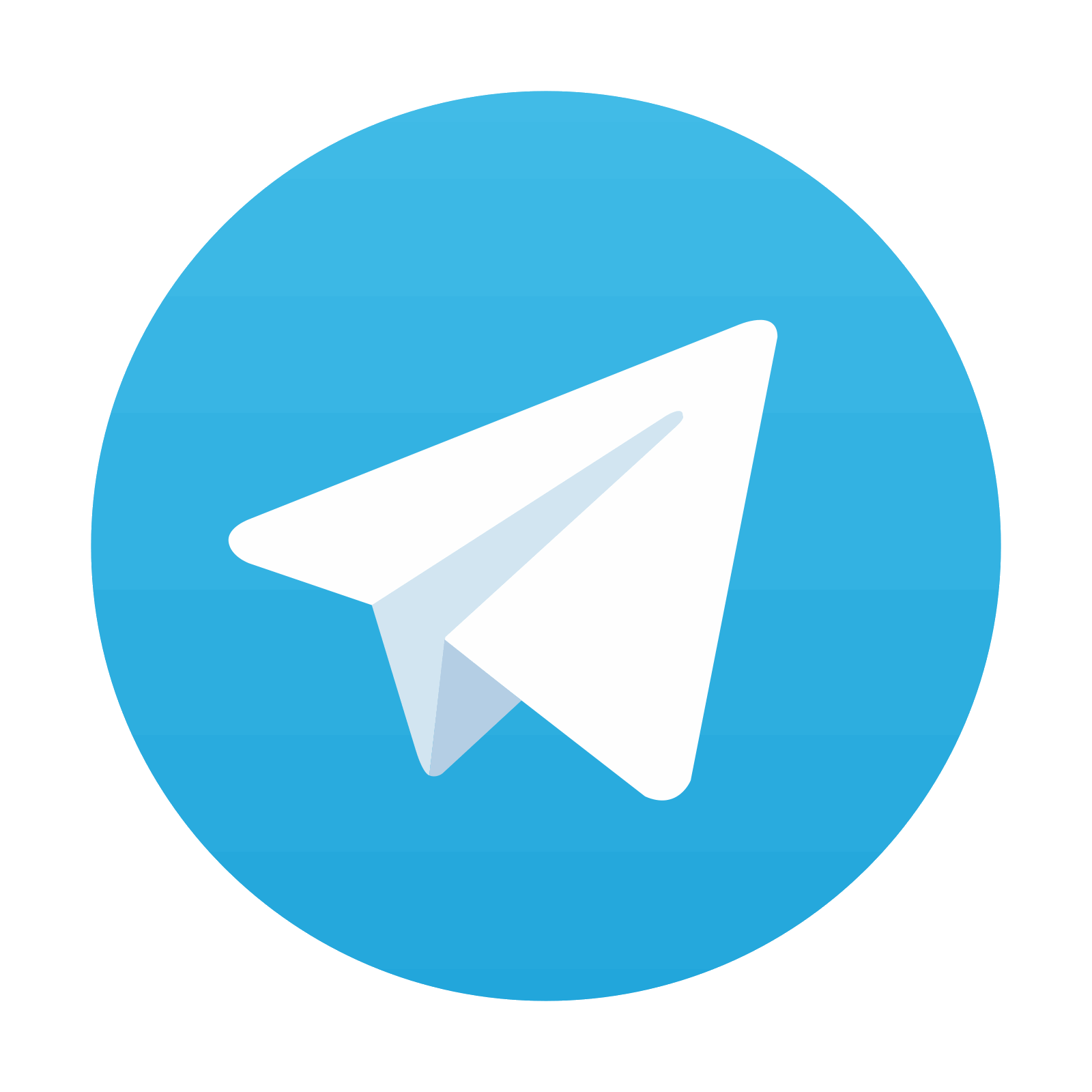
Stay updated, free articles. Join our Telegram channel
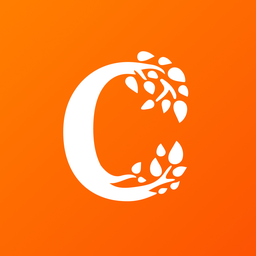
Full access? Get Clinical Tree
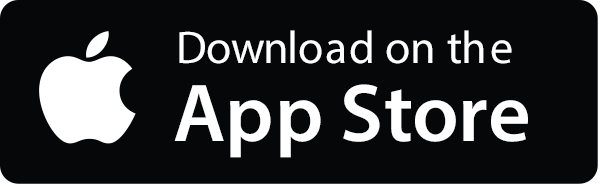
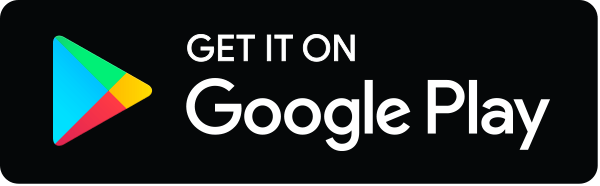