Abstract
Cryoablation is a safe and efficient energy for treating a variety of arrhythmic substrates at risk of atrioventricular (AV) block during ablation, including AV nodal reentrant tachycardia and septal pathways. It has been validated as an available option in atrial fibrillation ablation (balloon), typical flutter, and ventricular tachycardia. This chapter is describing the history, biophysics, and clinical use of cryoenergy in the electrophysiology laboratory.
Keywords
Cryoablation, Cryoenergy, Catheter ablation, Cryocatheter, Cryoballoon, Biophysics of cryoenergy
Key Points
- •
The biophysics and mechanisms of cryothermal injury comprise the following general phases: freeze/thaw, hemorrhage and inflammation, replacement fibrosis, and apoptosis.
- •
Cryoablation lesion size is determined by refrigerant flow rate, electrode size, electrode pressure, electrode orientation, duration of energy delivery, and electrode temperature.
- •
Advantages of cryoablation include the ability to titrate temperature/duration to produce reversible lesions before permanent tissue destruction (cryomapping), decreased risk of thromboembolism, superior catheter stability, and lesser risk of injury to vascular structures.
- •
Cryoablation has been applied clinically to a variety of arrhythmic substrates including, atrioventricular (AV) nodal ablation, AV nodal reentrant tachycardia, mid- and paraseptal pathways, ventricular tachycardia, atrial flutter, and atrial fibrillation.
The introduction of percutaneous direct-current ablation over 30 years ago launched an era of interventional cardiac electrophysiology that transformed the management of cardiac arrhythmias. Direct-current ablation was later supplanted by radiofrequency (RF) energy, which offered a more attractive safety and efficacy profile. Transcatheter RF ablation was broadly disseminated as the procedure of choice, with expanding indications that paralleled the growing global experience and knowledge base. Although the benefits of RF ablation became widely appreciated, limitations were noted, such as thromboembolization, inadvertent collateral damage to surrounding vascular and electrical structures, and the inability to assess electrophysiologic effects before permanent lesion creation were appreciated.
The scientific community therefore persevered in its efforts to further improve patient safety and procedural outcomes by seeking alternative sources of energy and developing ablation systems capable of creating deeper, larger, and more contiguous lesions. It is within this context that cryothermal energy ablation emerged as an alternative treatment modality. With the first transcatheter procedure performed in humans at the Montreal Heart Institute in August 1998, the collective experience has increased exponentially over the last 20 years. Potential advantages were recognized, including an impressive safety record with decreased thrombogenic potential, improved catheter stability during cryoapplications, lesser propensity to damage vascular structures, the ability to produce reversible electrophysiologic effects before permanent lesion creation, and decreased levels of pain perceived by patients.
The purpose of this chapter is to provide the clinical electrophysiologist, trainee, and cardiologist with a solid understanding of the field of cryoablation, beginning with a brief historical overview, discussion of biophysics, and depiction of the components of a transvenous catheter cryoenergy delivery system. Advantages and limitations of cryoablation are reviewed, and current clinical applications are discussed.
History of Cryothermal Energy Use in Cardiovascular Medicine
Cryotherapy, or the use of cold temperatures to elicit a specific tissue response, has a long history of effective medical use. The concept of cooling to treat medical disorders dates back to the ancient Egyptians, who described the use of hypothermic therapy between 3000 and 1600 bc . In the mid-1800s, iced saline was used for the treatment of carcinomas of the cervix and breast, followed by the topical use of liquid oxygen, nitrogen, and hydrogen for superficial carcinomas in the early 1900s. In 1948 Hass and coworkers first described predictable controlled myocardial lesions with cryoenergy using carbon dioxide as a refrigerant. In 1964 Lister and coworkers first described the application of cryoenergy to the cardiac conduction tissue by suturing a 4-mm U-shaped silver tube delivering cold energy near the bundle of His. Progressive but reversible high-grade atrioventricular (AV) block was demonstrated. Thus although not novel as an energy modality, harnessing cryoenergy into a steerable transcatheter format represents a more recent landmark in the history of arrhythmia therapy. Table 4.1 summarizes key historical landmarks in the development of a transvenous cryoablation system for cardiac arrhythmias.
Year | Authors (Ref. No.) | Contribution |
---|---|---|
1948–1951 | Hass and colleagues | Cryothermal myocardial lesions |
1963 | Cooper and Lee | Cryosurgical apparatus development |
1964 | Lister and coworkers | Cryothermal energy used to retard conductive tissue with evidence of reversibility |
1977 | Harrison and coworkers | Surgical application of cryothermal energy by handheld probe |
1991 | Gillette and coworkers | Percutaneous application of cryothermal energy by transvenous catheter in animals |
1998 | Dubuc and coworkers | Use of steerable cryocatheter system with pacing and recording electrodes |
2001 | Dubuc and coworkers | Percutaneous transvenous catheter cryoablation in humans |
The modern era of cryosurgery was initiated by Cooper and Lee when vacuum-insulated cryosurgical probes cooled by liquid nitrogen (−196°C) were introduced in the early 1960s. Evolving from Cooper’s apparatus, in which cooling was produced by a liquid-to-gas phase change in nitrogen, novel cryoprobes were subsequently developed using the Peltier effect (i.e., thermoelectric cooling by passing a direct current through dissimilar metal junctions) or cooling by the Joule–Thomson effect (i.e., cooling from the expansion of a highly compressed nonideal gas into a region of low pressure). Whereas the thermoelectric method offered limited thermal efficiency, the Joule–Thomson effect was relatively efficacious and spurred the development of diverse instruments (thin needle-like probes, clamp devices, catheter probes, and balloon structures), which in turn expanded the applications of cryoenergy for the treatment of a wide spectrum of dermatologic, prostatic, hepatic, gynecologic, ophthalmologic, neurologic, and oncologic disorders.
In the 1970s the rigid handheld cryothermal ablation probes were adapted for cardiac surgical applications as an alternative to open surgical dissection for the treatment of refractory arrhythmic substrates. In 1977 separate reports from Harrison and coworkers and Gallagher and coworkers demonstrated the efficacy of cryothermal ablation for supraventricular arrhythmic substrates. Under cardiopulmonary bypass, Harrison and coworkers produced complete AV nodal block using a handheld bipolar electrode probe by cooling the His bundle site to −55°C to −60°C for 90 to 120 seconds in 20 dogs; they also achieved AV block in three patients with refractory supraventricular tachycardia. Long-term follow-up on a larger series was later reported, with AV block successfully achieved in 17 of 22 patients. That same year, Gallagher and coworkers reported the first two cases of successful cryosurgical accessory pathway ablation. Following these early reports, several case series were published, including those targeting AV nodal reentrant tachycardia (AVNRT) and other arrhythmias with rapid AV conduction with the objective of slowing but preserving nodal conduction. In addition, reports were also published on novel approaches obviating the need for extracorporeal bypass (i.e., ablation within the coronary sinus or right coronary fossa, or through the use of dual atriotomy incisions), and targeting selected patients with refractory ventricular arrhythmias (often as an adjunct to more extensive surgery including aneurysmectomy, subendocardial resection, encircling endocardial ventriculotomy, coronary artery bypass grafting, and valvular replacement). Surgical cryoablation has also been described for less common arrhythmias including nodoventricular tachycardia, sinoatrial reentrant tachycardia, disabling ventricular bigeminy, bundle branch reentry tachycardia, and fetal malignant tachyarrhythmias.
Although insights gained from the extensive cryosurgical experience invaluably contributed to the conceptualization of the modern transvenous catheter cryoablation system, significant engineering advances were required to achieve the safe and effective delivery of a pressurized cryorefrigerant to the tip of a steerable percutaneous catheter. Gillette and coworkers reported the first animal study using a transvenous cryocatheter in 1991. In five miniature swine, complete AV block was produced with an 11-F cryocatheter cooled by pressurized nitrous oxide. Although feasibility of transcatheter cryolesion formation was demonstrated, modest success was attributed to limited maneuverability as well as the lack of recording electrodes (i.e., cryocatheter positioning required the aid of a second catheter to record local signals). Transcatheter cryoablation was revived several years later, ultimately leading to clinical use. In 1998 the first animal experiment using a steerable cryocatheter with integrated recording and pacing electrodes was reported. This 9-F catheter system used halocarbon 502 (Freon) as a refrigerant. Chronic histology was later characterized, with sharply demarcated ultrastructurally intact lesions devoid of thrombus. These and other preclinical studies contributed importantly to the understanding of the impact of cooling rate and catheter-tip temperature on tissue effects.
Biophysics and Mechanisms of Cryothermal Energy Tissue Injury
The ultimate purpose of cryoablation is to freeze tissue in a discrete and focused fashion to destroy cells in a precisely targeted area. The application of cryothermal energy results in the formation of an ice ball. Cooling first occurs at the distal catheter tip in contact with endocardial tissue. Freezing then extends radially into the tissue, establishing a temperature gradient. The lowest temperature and fastest freezing rate are generated at the point of contact, with slower tissue cooling rates at the peripheral regions. The mechanisms of tissue damage are complex and still debated but involve freezing and thawing, hemorrhage and inflammation, replacement fibrosis, and apoptosis ( Fig. 4.1 ).

Tissue hypothermia causes cardiomyocytes to become less fluidic as metabolism slows, ion pumps to lose transport capabilities, and intracellular pH to become more acidic. These effects may be transient, depending on the interplay between temperature and duration. The shorter the exposure to a hypothermic insult and/or the warmer the temperature, the faster the cells recover. As a clinical correlate, this characteristic feature of cryoenergy permits functional assessment of putative ablation sites (i.e., cryomapping) without cellular destruction.
By contrast, the hallmark of permanent tissue injury induced by hypothermia is the formation of ice crystals. As cells are rapidly cooled to freezing temperatures, ice crystals are first formed within the extracellular matrix, and then intracellularly. The size of ice crystals and their density are dependent on the combination of the following: proximity to the cryoenergy source, local tissue temperature achieved, and rate of freezing. Initially, ice crystals are formed exclusively in the extracellular space as the tissue temperature drops below −15°C. Progressive cooling to below −40°C results in the formation of intracellular ice crystals. Although ice crystals are associated with mechanical cellular disruption, the predominant mechanism of cellular injury is biochemical. The formation of extracellular ice crystals results in the extracellular space becoming relative hypertonic. To reestablish osmotic equilibrium, there is a compensatory egress of water from the intracellular to the extracellular space, with subsequent cellular shrinkage, resulting in intracellular desiccation. Further, the newly established osmotic gradient precipitates a diffusion gradient between extracellular and intracellular spaces, resulting in the net movement of hydrogen (H) + ions out of the cell and the migration of solute ions into the cell. Concomitant increase in the intracellular saline concentration with a reduction in intracellular pH results in cellular protein damage, enzyme system impairment, and adverse effects on lipoprotein components of the plasma membrane. Of all the cytoplasmic components, the mitochondria are particularly sensitive and are the first structures to suffer irreversible damage.
Upon completion of the freezing phase, the tissue passively returns to body temperature resulting in a thawing effect. This second phase induces cellular damage through a combination of two mechanisms. First, recrystallization and coalescence of intracellular and extracellular ice crystals increase the osmotic damage and generate shear forces, which further disrupt tissue architecture. Second, restoration of microcirculatory function is associated with a hyperemic vascular response characterized by hemorrhage and inflammation (coagulation necrosis; Fig. 4.2 , A ). Specifically, blood vessel walls become porous, leading to increased capillary permeability, and subsequent interstitial edema. This vascular congestion, combined with endothelial injury, induces platelet aggregation and microthrombi formation, and culminates in vascular obliteration and ischemic cellular necrosis. As such, although the central region subjected to the coldest freezing temperatures undergoes direct cellular damage, the surrounding microvascular injury results in the extension of tissue destruction.

The final phase of cryoinjury begins concurrent to thawing and is characterized by reactive inflammation, followed by tissue repair and replacement fibrosis. Over the subsequent weeks, these processes culminate in the generation of a mature lesion (see Fig. 4.2 , B ), which has a distinct, well-circumscribed central region of dense fibrosis surrounded by a narrow border zone of variable cellular death (because of microvascular injury and apoptosis).
Cryoablation Technical Aspects
Console and Catheters
Although insights gained from the extensive cryosurgical experience invaluably contributed to the conceptualization of the modern transvenous catheter cryoablation system, a significant number of engineering advances were required to achieve safe and effective delivery of cryorefrigerant to the tip of a steerable percutaneous catheter. Although the system has undergone various modifications since its conception in the early 1990s, the basic design has remained constant. Specifically, the modern transvenous deflectable cryocatheter consists of a hollow shaft with a closed distal end containing a cooling electrode tip, integrated thermocouple device, and three proximal ring electrodes for recording and pacing ( Fig. 4.3 ). A central CryoConsole (Medtronic CryoCath LP, Pointe-Claire, QC, Canada) contains the cryorefrigerant fluid ( Fig. 4.4 ). The cooling liquid travels through the inner delivery lumen to the catheter tip, where the cryorefrigerant is pressurized and released. This accelerated liquid-to-gas phase change results in rapid cooling of the distal tip. The gas is then conducted away from the catheter tip through a second coaxial return lumen maintained under vacuum and evacuated in the hospital medical gas disposal system.


The console allows the operator two different modes of operation. The first is the cryomapping mode in which the tip is cooled to a temperature not lower than −30°C for a maximum of 80 seconds, so as to prevent irreversible tissue damage. The second mode is cryoablation, which results in cooling of the catheter tip to at least −75°C for a programmable period (nominally 4 minutes), producing the permanent lesion. The cryomapping mode can be used for an indefinite number of times before cryoablation. Cryoablation may be initiated at any time during a cryomapping application or, from the onset, if the operator wishes to forego the cryomapping function.
Although the core tenets of its design have remained largely unchanged, the transvenous cryoablation catheter has undergone significant evolutionary advances over the last decade. The refrigerant has progressed from halocarbon 502 (Freon) to Genetron AZ-20 to the current nitrous oxide–generating lower catheter temperatures and faster freezing rates. Catheter diameter has been reduced from 9 F to 7 F, while larger electrode-tip sizes were introduced (4, 6, and 8 mm; Fig. 4.5 ). Steering mechanisms were developed and refined. Finally, with the introduction of innovative catheter configurations (focal, linear, circular, and balloon-based apparatuses), diverse clinical applications have been explored. Thus within a relatively brief time frame, the initial 9 F focal cryoablation catheter with slow cooling and a temperature limit of −50°C was transformed into the modern 7 F catheter with rapid cooling and achievable temperatures below −80°C.

In addition, an expandable 23-mm (currently abandoned) and 28-mm diameter cryoballoon (CB) catheter (Arctic Front; Medtronic CryoCath LP) was specifically designed to isolate pulmonary veins (PVs) in patients with atrial fibrillation (AF; Fig. 4.6 ). The basic design and function of the CB catheter differ from the focal catheter in three major respects. First, instead of a rigid cooling electrode tip, the distal CB catheter consists of dual polyurethane and polyester balloons. Second, the CB is larger than the focal catheter (10.5-F outer diameter) and requires the use of a 15 F (outer diameter) deflectable delivery sheath (FlexCath; Medtronic CryoCath LP). Third, the CB is deployed using an over-the-wire technique with a central lumen that permits a guidewire for positioning/support, or a small-diameter circular diagnostic catheter for monitoring of PV potentials, as well as contrast injection to ensure adequate positioning. Moreover, the CB catheter underwent an evolutionary revision ( Fig. 4.7 ). In the first-generation catheter, 6.2 L per minute of vapor was sprayed to the distal face of the balloon through four jets positioned at 90 degrees from one another, slightly distal to the CB equator. This resulted in a band of optimal cooling that was relatively equatorial and nonuniform between the two CB sizes. Specifically, given the identical refrigerant delivery mechanisms, the larger surface area associated with the 28-mm CB meant that the cryorefrigerant was delivered in a relatively less concentrated manner, resulting in heterogeneous cooling across the anterior aspect of the CB. The second-generation catheter refined the design by repositioning the jets more distally, increasing the number of jets to eight, and increasing the flow rate in the larger CB catheter to ensure more uniform ablative temperatures around the entirety of the distal surface of the CB.


Determinants of Cryoablation Lesion Size
During catheter cryoablation, tissue temperatures follow a monoexponential decline toward steady-state values about the ablation electrode ( Fig. 4.8 ). The size of catheter-based cryoablation lesions is dependent on many factors ( Table 4.2 ). Analogous to electrical current for RF ablation, the refrigerant is the mediator of thermal change in cryoablation systems. Higher flow rates of refrigerant are capable of extracting more heat from the tissue, and therefore lesion sizes are increased. The more the temperature drop is abrupt and deep, the more the induced lesion is significant. In addition, refrigerants differ in their capacity to extract heat based on their physical properties and physical phase delivered to the electrode tip. For example, a colder gas phase of refrigerant may well produce a smaller lesion than a liquid refrigerant undergoing a phase change within the electrode at a warmer temperature. Larger electrode sizes appear tied to larger lesions by way of allowing greater refrigerant flow rates. Lesion surface areas produced by 8-mm catheters are, on average, 92 mm 2 larger (177%) than by 4-mm catheters and 72 mm 2 larger (101%) than by 6-mm catheters. The 8- and 6-mm catheters yield mean lesion volumes 253 mm 3 (248%) and 116 mm 3 (114%) larger than 4-mm catheters, respectively. Lesion sizes also increase with greater electrode contact pressure and with greater electrode surface area in contact with the tissue to allow greater heat extraction from the tissue and less from the local blood pool. Electrode orientations that are parallel with the tissue therefore produce larger lesions than perpendicular orientations because of greater thermal coupling with the tissue. Convective warming of electrode and tissue by local blood flow has a detrimental effect on lesion formation. In experimental preparations, simulated blood flow over cryoablation electrodes may reduce lesion volume by 75% compared with the absence of blood flow. Even under strictly controlled experimental conditions, electrode temperature is an imperfect predictor of lesion size ( Fig. 4.9 ). Because active cooling occurs within the electrode and near the embedded thermocouple, electrode temperature is insensitive to other factors critical to lesion formation such as convective warming, contact pressure, and electrode orientation. In addition, maximal electrode cooling may occur in the absence of any tissue contact. This differs from RF ablation in which the electrode is passively heated from contact with the tissue. In isolated tissue experiments, lesion dimensions were increased by prolonging energy delivery or by repeating the freeze–thaw cycle when compared with single 2.5-minute applications.

Factor | Effect On Lesion Size |
---|---|
Refrigerant flow rate | Increased flow increases lesion size |
Electrode size | Increased electrode size allows greater refrigerant flow rates |
Tissue contact | Increased contact pressure increases lesion size |
Electrode orientation | Larger lesion sizes with horizontal (parallel) electrode orientation to tissue |
Convective warming | Blood flow over electrode/tissue reduces lesion size |
Electrode temperature | Colder temperature creates larger lesion a |
Duration of energy application | Longer delivery produces larger lesion |

Cryoablation versus Radiofrequency Ablation
In comparison with RF catheter ablation, lesions associated with focal cryothermal ablation have a smaller surface area caused, in part, by cryoadhesion-induced loss of the brushing effect, with no difference in lesion depth. Histologically, cryothermy results in a dense, homogeneous fibrosis that is clearly demarcated from normal myocardium ( Fig. 4.10 , A , right ). By contrast, the hyperthermic injury induced by RF energy results in diffuse cellular destruction characterized by intralesional hemorrhage and ragged edges less clearly demarcated from normal myocardium (see Fig. 4.10 , A, left ). In addition, although devitalized, lesions produced by cryothermal ablation are associated with preservation of ultrastructural integrity, an observation that is attributed to the remarkable resilience of fibroblasts and collagen fibers to hypothermia. Preservation of tissue ultrastructural integrity should theoretically result in a lower risk of myocardial perforation, esophageal injury, and aneurysmal dilation. It may be speculated that lesions produced with cryoablation should be less arrhythmogenic (given that distinct border zones are less susceptible to spontaneous depolarization) and associated with a lower incidence of venous or arterial stenosis (given the minimal tissue contraction observed with lesion healing). Thermal profiles for cryoablation and RF ablation lesions obtained by infrared thermography are shown in Fig. 4.10 , D .

Some factors influencing lesion size for catheter cryoablation are also critical to RF ablation, whereas others may have opposite effects for the two energy sources ( Fig. 4.11 ). Both modalities benefit from enhanced tissue-contact pressure and larger electrode sizes if the larger electrode allows greater refrigerant flow or electrical current to be delivered. Creation of larger lesions with cryoablation depends on conditions such as convective thermal effects and electrode orientation. For RF ablation, convective cooling of the electrode by local blood flow can enhance power delivery. For cryoablation, local blood flow can only be detrimental by warming the electrode and tissue. For cryoablation and noncooled RF ablation, an electrode orientation parallel to the tissue enhances lesion size. For irrigated RF catheters, a parallel electrode orientation reduces lesion size. Simultaneously applying standard RF and cryothermal energy through the same catheter may produce lesions of similar dimension to irrigated RF ablation.

Cryomapping and Cryoablation Delivery
Standard ablation with the CryoCath system consists of advancing a steerable quadripolar catheter to the region of interest. The ablation target is identified with mapping techniques similar to RF ablation procedures. Once the target is identified, the operator may select between cryomapping and cryoablation modes. The cryomapping mode is typically used before cryoablation when the arrhythmia substrate is in the vicinity of the AV node/His–Purkinje conduction system. This mode consists of the use of milder freezing temperatures at the target lesion site to assess clinical effect (efficacy mapping) and ensure the absence of adverse clinical outcomes (safety mapping). The operator may choose to apply cryoablation directly if the region is deemed safe or at some distance from the conduction system. It is important to note that although safety and efficacy mapping may be realized through the use of the cryomapping feature, a dynamic cryomapping process will naturally occur over the course of a cryoapplication as the hypothermic wave front spreads centrifugally from the catheter tip to the surrounding tissue. In effect, the cooling of cells with reversible electrophysiologic effects (e.g., cryoprobe temperature, 0°C to −30°C; tissue temperature, 0°C to −5°C) necessarily precedes irreversible tissue destruction (e.g., at temperatures of <−50°C to −60°C). Thus vigilance must be maintained throughout the cryoapplication when treating critical substrates (e.g., perinodal), because tissues not initially affected have the potential to undergo reversible suppression as the cryolesion continues to expand during cryoablation.
When temperatures reach −20°C, electrical noise appears on the distal electrode pair, with loss of the local electrogram signal because of the formation of the ice ball. This electrical noise resolves once the temperature warms to over −20°C. During the time that temperatures remain colder than −20°C, the catheter adheres to the cardiac endocardial tissue and, therefore allows the operator to perform programmed stimulation to confirm safety and/or efficacy without concern for catheter dislodgment. In the event of an undesirable effect, prompt termination of the application usually results in complete recovery within seconds after rewarming, with no permanent effect. If desired effects are confirmed, cryoablation is typically maintained for 4 minutes, largely because preclinical studies based on correlation between histopathologic analyses and visualization of ice ball formation demonstrated that the lesion increases in size during the first 2 to 3 minutes and reaches a plateau thereafter ( Fig. 4.12 , A–C ). However, chlorofluorocarbon agents have since been replaced by a more potent refrigerant, currently nitrous oxide (N 2 O), which produces a faster drop in temperatures and lower absolute values. The standard 4-minute application has been questioned, with preclinical studies of focal cryocatheter and CB ablation suggesting that shorter application times (e.g., 2-minutes) suffice.

Although one application is typically sufficient to create permanent effects on conduction, double freeze–thaw cycles or multiple applications may be performed if desired or required to create more homogeneous necrosis.
When performing PV isolation (PVI) for the treatment of AF with cryoenergy, the delivery tool is different and based on a balloon technology. The standard technique with the Arctic Front catheter consists of inserting a guidewire in a PV, advancing the catheter over the wire to the desired location, inflating the balloon, assessing tissue contact by injecting contrast through the catheter’s central lumen demonstrating venous occlusion, in the absence of leaks, and applying 2 to 4 minutes of cryoablation.
Clinical Advantages of Cryothermal Energy for Catheter Ablation
Theoretical advantages of cryothermal over RF ablation are summarized in Table 4.3 and include reversibility, catheter stability, minimal risk of thromboembolism, safety near vascular structures, and decreased pain perception.
Advantages | Clinical Implications |
---|---|
Catheter adhesiveness | Greater catheter stability Programmed stimulation may be performed during ablation Avoidance of brushing effects |
Homogeneous sharply demarcated lesion | Less arrhythmogenic More controllable titration of lesion size |
Preservation of ultrastructural integrity | Decreased risk of thrombus formation Absence of aneurysmal dilation or rupture |
Reversible suppression of conduction tissue | Prediction of successful site Avoidance of unwanted lesions Ablation of high-risk substrates |
Lesion limited by warming blood flow | Safety to nearby epicardial coronary arteries |
Visualization by ultrasound | Real-time monitoring Confirmation of endocardial contact Defining optimal freezing parameters |
Pain-free ablation | Discomfort minimized under conscious sedation |
Reversible Effects
As previously discussed, one of the most exciting and truly remarkable characteristics of cryothermal energy is the ability to dynamically and prospectively assess the safety and efficacy of a potential ablation lesion site, because a period of reversible electrophysiologic tissue inhibition obligatorily precedes permanent tissue destruction (a process that can be dynamically manipulated by varying the temperature and/or time of application). Although extreme freezing (i.e., tissue temperatures colder than −50°C) results in near instantaneous permanent tissue injury, a functional effect may be obtained at sublethal temperatures (i.e., −10°C to −25°C), with complete recovery of all electrophysiologic properties and no histologically identifiable damage ( Fig. 4.13 , A–D ). Cryomapping is not only theoretically possible, but the broad temperature/time window between reversible and irreversible effects renders this feature readily clinically applicable. Thus by identifying the desired substrate before definitive ablation, the appropriate catheter placement site may be confirmed to be efficacious (i.e., efficacy cryomapping) and/or safe (i.e., safety cryomapping). Reversible cryomapping may be of particular importance when ablating arrhythmogenic substrates located near critical sites such as the AV node, where a missed target lesion may have major consequences. Reversibility observed with cryothermal energy contrasts starkly with RF energy. With RF ablation, hyperthermal tissue injury leading to reversible loss of excitability occurs at a median tissue temperature of 48°C, whereas irreversible tissue destruction occurs at tissue temperatures greater than 50°C. The RF reversibility window is therefore too narrow for safe clinical applications.
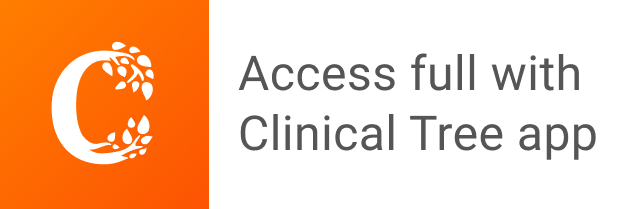