Hypoxic pulmonary vasoconstriction Mechanisms of hypoxic pulmonary vasoconstriction Remodeling of the pulmonary circulation with prolonged exposure Effect of HPV on exercise performance Preservation of tissue oxygenation at high altitude depends not only on the efficiency of gas exchange (Chapter 8) and maintenance of adequate arterial oxygen content (Chapter 10), but also on the ability to deliver oxygenated blood to the tissues. It is for this reason that the cardiovascular system serves an essential link in the transport of oxygen from the air to the mitochondria, and as a result, plays an important role in acclimatization and adaptation to high altitude. The objective of this chapter is to discuss cardiovascular responses to high altitude hypoxia and how these responses impact global cardiovascular performance, review limitations and important gaps in knowledge, and emphasize future directions to further our understanding of the cardiovascular system at high altitude. While the literature on this topic has considerable overlap between cardiac function at rest and with exercise, this chapter will focus primarily on cardiac function at rest, while the bulk of the discussion of changes seen with exercise is considered in Chapter 18. The core function of the cardiovascular system is to deliver oxygenated blood to the tissues. For that reason, any discussion of changes in cardiac function at high altitude must begin with consideration of changes in cardiac output. Experiments early in the last century showed a general pattern of a rise in cardiac output with acute exposure to high altitude, followed by a fall to at or near sea-level values with acclimatization. Barcroft et al. (1923) reported an increase in cardiac output at rest soon after reaching high altitude using an indirect Fick technique at Cerro de Pasco in the Peruvian Andes (4330 m). In a series of elegant experiments using acetylene rebreathing at Pikes Peak, Grollman (1930) reported elevated cardiac output at rest soon after reaching high altitude, with a maximum value observed approximately five days later. By day 12, cardiac output had returned to its sea-level value. Similar changes in cardiac output were observed by Christensen and Forbes (1937) during the International High Altitude Expedition to Chile, by Klausen (1966) at the Barcroft laboratory (3800 m) and Vogel et al. (1967) (Figure 11.1) on Pikes Peak at 4300 m. However, some groups found that following several days of acclimatization, cardiac output was slightly reduced compared to sea-level values (Balasubramanian et al. 1978; Hoon et al. 1977; Sime et al. 1974; Wolfel et al. 1994), possibly secondary to an increase in hemoglobin mass and oxygen carrying capacity. Importantly, cardiac function, measured via cardiac catheterization, is maintained even when humans are decompressed in a hypobaric chamber down to an inspired PO2 of 43 mmHg, equivalent to that of the Everest summit. Together, these data present a consistent pattern whereby cardiac output increases acutely on exposure to high altitude by approximately ∼50%, maintaining convective oxygen delivery in the face of reduced arterial oxygen content, and returns to or slightly below sea level values as arterial oxygen content increases with acclimatization. Figure 11.1Cardiac output (solid line), mean systemic arterial pressure (dashed line), and calculated peripheral resistance (dotted line) during acute exposure to a simulated altitude of 2000 ft (610 m), 11,000 ft (3353 m), and 15,000 ft (4572 m). Measurements were made on 16 subjects after 10, 20, 30, and 40 hours at each altitude. The results from the different altitude exposures were pooled. Mean ± SE indicated by vertical bars (1 dyne = 10−5 N). (Source: Vogel and Harris 1967.) Further understanding of these changes in cardiac output comes from looking at the two parameters upon which it is dependent, heart rate and stroke volume. Acute hypoxia causes a rise in heart rate proportional to the decline in ambient PO2 (Koller et al. 1988; Vogel and Harris 1967). At simulated altitudes of 4000–4600 m where acute exposure depresses the arterial PO2 to 40–45 mmHg, resting heart rates increase by 40–50% above sea-level values (Kontos et al. 1967; Vogel and Harris 1967). The cause of tachycardia is unlikely due to a direct effect of hypoxia on intrinsic sinus node function, but rather due to changes in autonomic nervous system function. As a result, many studies in this field utilize the systemic administration of pharmacological agents, such as propranolol (beta-blocker, sympathetic blockade), atropine, and glycopyrrolate (anticholinergics, parasympathetic blockade) to manipulate autonomic control of heart rate. Hypoxemia activates the peripheral chemoreceptors (Marshall 1994), which leads to elevated muscle sympathetic nerve activity (Hansen and Sander 2003; Rowell et al. 1989; Somers et al. 1988), plasma urinary catecholamines, and circulating epinephrine and norepinephrine (Richalet 1990). However, while hypoxemia clearly causes profound sympathetic activation, the rise in resting heart is not solely dependent on β-sympathetic activation. For example, under conditions of isolated β-sympathetic blockade with propranolol, the heart rate response to hypoxia practically parallels the control condition when β-sympathetic control is present (Hopkins et al. 2003; Koller et al. 1988; Richardson et al. 1967; Siebenmann et al. 2015). The heart rate response is also present when the parasympathetic arm of this reflex is blocked (Hopkins et al. 2003; Siebenmann et al. 2015), but completely abolished when both muscarinic and β-receptors are inhibited (Siebenmann et al. 2015). Therefore, in response to hypoxemia at rest, the cardiovascular system is capable of augmenting heart rate independently via either arm of the autonomic reflex in order to appropriately adjust cardiac output to maintain oxygen delivery. Vagal modification via pulmonary stretch receptors appears intuitive as the mechanism of parasympathetic withdrawal, as RR shortening occurs during inspiration (Brown et al. 1993). Yet, controlling ventilation in both normoxia and hypoxia to values obtained during spontaneous breathing does not attenuate the acute vagally mediated increase in heart rate (Siebenmann et al. 2019). Potential involvement of the aortic arch chemoreceptors was suggested as a possible mechanism by the investigators. Challenging future experiments will be necessary to investigate this possibility. Heart rate remains elevated despite acclimatization to high altitude. While, sympathoexcitation persists (Hansen and Sander 2003), this arm of the autonomic reflex still seems to play a minor role, at least at moderate altitudes, as the rise in heart rate is unaffected by sympathetic blockade after two weeks at 3454 m (Siebenmann et al. 2017). Several studies (Hughson et al. 1994; Siebenmann et al. 2017; Wolfel et al. 1994) have shown that parasympathetic blockade abolishes the rise in heart rate after acclimatization, which suggests parasympathetic control dominates the hypoxia-induced acceleration of resting heart rate in acclimatized lowlanders (Figure 11.2). One possible explanation for the observation of high sympathetic activation, but minimal role in heart rate control is that persistent sympathetic stimulation downregulates cardiac β-adrenergic receptor sensitivity and, thus, the effectiveness of sympathetic activation on the heart (Kacimi et al. 1992; Richalet et al. 1988). Figure 11.2Changes in resting heart rate with high altitude exposure. Each filled circle represents values for an individual subject, while the short horizontal lines denote the average values at sea level (SL) and high altitude (HA), respectively. P values are for the comparison between SL and HA within the respective drug condition. CONT, control; GLYC, glycopyrrolate; PROP, propranolol; PROP+GLYC, propranolol and glycopyrrolate in combination. (Source: Siebenmann et al. 2017.) Overall, it seems that under conditions of acute hypoxia, heart rate and thus cardiac output can be elevated via sympathetic activation or withdrawal of parasympathetic tone. This level of redundancy likely reflects the primacy of this response in maintaining oxygen delivery. With short-term acclimatization, cardiac β-adrenergic receptor sensitivity is downregulated and heart rate remains elevated due to mild parasympathetic withdrawal. An important caveat to this interpretation, however, is that the studies noted above used varying methodologies including a variety of altitudes or inspired oxygen fractions, as well as small sample sizes and short periods of acclimatization (i.e., a few weeks) to moderate altitudes. Indeed, heart rate falls consistently with sympathetic blockade in long-term (>2 years) Han Chinese residents at 3658 m (Zhuang et al. 1993). Moreover, the increase in heart rate with parasympathetic blockade is substantially greater (sea level, 61 ± 63 to 114 ± 66 bpm; acclimatization, 73 ± 64 to 153 ± 64 bpm) after nine weeks residence at >5260 m than sea level (Boushel et al. 2001), implying that vagal control may mask high sympathetic activation at very high altitudes. Stroke volume is relatively unaffected by acute hypoxemia, but declines over time as acclimatization proceeds (Boussuges et al. 2000; Reeves et al. 1987; Siebenmann et al. 2013; Stembridge et al. 2019; Vogel et al. 1967). As noted above, the persistent increase in heart rate maintains cardiac output despite this reduction in stroke volume. The reduced stroke volume seen with acclimatization could theoretically be caused by impaired myocardial contractility, reduced cardiac filling, or increased afterload on the right or left ventricle due to increases in pulmonary artery or aortic pressure, respectively. Each factor is considered below. Initial evidence that myocardial contractility was preserved at high altitude came from Operation Everest II (Suarez et al. 1987), where two-dimensional echocardiography demonstrated that ejection fraction, the ratio of peak systolic pressure to end-systolic volume, and mean normalized systolic volume at rest were all sustained at a barometric pressure of 282 mmHg, corresponding to an altitude of about 8000 m. The myocardium extracts a large proportion of oxygen from the coronary arterial blood, with the result that the venous PO2 in the heart has one of the lowest values of all organs in the body. Yet, with an acute fall in arterial PO2, pronounced coronary artery vasodilation maintains myocardial oxygen delivery (Kaufmann et al. 2001; Regan et al. 1963; Wyss et al. 2003) and limits the direct effect of hypoxia on myocardial function. While global measurements confirm normal contractile function (Reeves et al. 1987), subtle intrinsic myocardial adaptations highlight how the human heart tries to support ejection fraction when filling pressure and presumably Frank-Starling-mediated contractility are reduced during acclimatization. In summary, diastolic relaxation may be supported by a more rapid left ventricular untwisting velocity, while systolic function is supported by augmented left ventricular twist mechanics (Stembridge and Levine 2019). While alterations in left ventricular twist has been proposed to represent impaired function due to subendocardial hypoxemia (Osculati et al. 2016), experimental data have disproved this hypothesis. Using the β1-adrenergic receptor antagonist esmolol, Williams et al. (2019) documented that augmentation of left ventricular basal and apical rotation (i.e., twist mechanics) is due to both an β1-adrenergic receptor dependent and independent mechanism, while a regional strain-based analysis of the myocardium suggested there is no endocardial dysfunction. Decreased venous return could result from either increased heart rate and thus a shorter time for ventricular filling or a reduction of circulating blood volume, or both. The influence of heart rate is likely minimal at best, as the decrease in cardiac filling time with modest increases in resting heart rate is typically not sufficient to impair ventricular filling in individuals with otherwise normal cardiac function. Indeed, heart rate is similarly elevated with acute hypoxia when resting stroke volume is normal (Vogel and Harris 1967). In addition, lowering of HR by β-blockade at altitude does not restore resting stroke volume (Wolfel et al. 1994). During Operation Everest II, it was possible to measure both mean right atrial pressure, a marker of right ventricular filling pressure, and pulmonary artery wedge pressure, a marker of left ventricular filling pressure. Both measurements tended to fall alongside stroke volume as simulated altitude increased (Reeves et al. 1987). The fall in left ventricular filling pressure has been attributed to the well-known reduction in circulating blood volume due to the loss in plasma volume with short term (four weeks) acclimatization, as discussed in Chapter 13 (Alexander et al. 1967; Grover et al. 1976; Robach et al. 2000; Ryan et al. 2014). Restoration of blood volume via acute plasma volume expansion normalizes left ventricular filling and restores resting stroke volume to sea-level values (Stembridge et al. 2019), at least with short-term exposure to moderate altitudes (3454–3800 m, Figure 11.3). Interestingly, at higher altitudes (> 5000 m), acute plasma volume expansion seems to have a limited impact on resting stroke volume (Calbet et al. 2004). Figure 11.3Heart rate, left ventricular stroke volume (LV SV), and cardiac output at sea level (SL) and at high altitude (HA) following plasma volume expansion (HA-PVX), sildenafil administration (HA-SIL), and combined plasma volume expansion and sildenafil administration (HA-PVX-SIL). Statistical significance values reported on the figures are from the two separate one-way ANOVA tests run to compare SL with HA and HA-PVX and SL with HA-SIL and HA-PVX-SIL. P values in smaller font refer to the post hoc tests where each condition was compared to sea level, and effect sizes (Cohen’s d) and 5–95% confidence intervals (CI) are also reported. Each symbol represents the same individual across all conditions. (Source: Stembridge et al. 2019.) As discussed in greater detail later in this chapter and again in Chapter 22, acute alveolar hypoxia leads to constriction of pulmonary arterioles, thereby increasing pulmonary vascular resistance, pulmonary artery pressure, and thus, afterload of the right ventricle. The effect of such pulmonary vasoconstriction on stroke volume is likely limited. Administration of the pulmonary vasodilator sildenafil, for example, reduces pulmonary artery systolic pressure at high altitude, but leads to only minor improvements in stroke volume (Stembridge et al. 2019). Moreover, when pulmonary artery pressure is grossly elevated in patients with high altitude pulmonary edema (Chapter 22), measures of left ventricular function remain unchanged from sea-level values, even in subjects with increased resting right ventricle pressure gradients of 43 ± 9 mmHg (Bernheim et al. 2007). Despite the fact that hypoxic myocardium is prone to arrhythmia (Josephson and Wellens 1984) and individuals climbing at the extremes of elevation can experience arterial PO2 values as low as 25–30 mmHg near the summit of Mount Everest (Grocott et al. 2009; West et al. 1983), clinically significant arrhythmias are overall uncommon, although other changes in conduction can be seen. An extensive study of cardiac electrical conduction was carried out during the 1981 American Medical Research Expedition to Everest (AMREE) (Karliner et al. 1985) when recordings were made at sea level, 5400 m, 6300 m, and again at sea level. A total of 19 subjects were studied, although complete data were not obtained from all. Resting heart rate increased from a mean of 57 beats minute−1 at sea level to 70 beats minute−1 at 5400 m and 80 beats minute−1 at 6300 m. The amplitude of the P-wave in standard lead 2 of the electrocardiogram increased by more than 40% from sea level to 6300 m, consistent with right atrial enlargement. Right axis deviation of the QRS axis was seen, with the mean frontal plane QRS axis increasing from +64° to +78° at 5400 m and +85° at 6300 m. Three subjects showed abnormalities of right bundle branch conduction at the highest altitude and three others showed changes consistent with right ventricular hypertrophy, including posterior displacement of the QRS vector in the horizontal plane. Seven subjects developed flattened T waves and four showed T-wave inversions (Figure 11.4). All the changes returned to normal in tracings obtained at sea level after the expedition. Figure 11.4Twelve-lead electrocardiogram obtained from a single individual at Camp 2 (6300 m) and about three months after return to sea level. Sinus tachycardia and diffuse T-wave flattening are present at altitude; the T waves in leads V2 and V3 exhibit terminal inversion. (Source: Karliner et al. 1985.) Other investigators have reported similar findings in acclimatized lowlanders, though generally in smaller numbers of subjects or at lower altitudes. Milledge (1963) made measurements during the 1960–61 Silver Hut Expedition and reported data on subjects who spent several months at an altitude of 5800 m. He found T-wave inversions on the right precordial leads in six subjects and left precordial T-wave inversion in two subjects, neither of which resolved with oxygen breathing. Das et al. (1983) reported on more than 40 subjects who were rapidly transported to either 3200 m or 3771 m. There was a tendency for rightward axis deviation which, interestingly, tended to resolve in most subjects after 10 days at high altitude. A particularly remarkable measurement was made by a single member of the 1975 Chinese ascent of Mount Everest who laid down on the summit under the newly erected tripod while a standard lead 1 signal was transmitted by telemetry to base camp. No changes were noted from sea level to 8848 m and back again (Shi et al. 1980). Other electrocardiographic studies at high altitude include those made by Peñaloza and Echevarria (1957), Jackson and Davies (1960), Aigner et al. (1980), Malconian et al. (1990), Chandrashekhar et al. (1992), and Halperin et al. (1998). In the AMREE study noted previously, only one subject had premature ventricular contractions at an altitude of 5300 m, while another climber showed premature atrial contractions at 6300 m (Karliner et al. 1985). One subject on the 1960–61 Silver Hut Expedition showed premature ventricular contractions after exercise at an altitude of 5800 m (Figure 11.5), but no other member of the expedition showed any dysrhythmia (Milledge 1963). Occasional premature ventricular contractions and premature atrial contractions have been observed by others (Cummings and Lysgaard 1981). Figure 11.5Electrocardiogram showing premature ventricular contractions occurring after exercise at 5800 m. (Source: Milledge 1963.) One limitation of all of the studies mentioned above, however, is that they are subject to a significant sampling bias related to the short time frame of electrocardiogram (ECG) acquisition. Better information can now be obtained using implantable loop recorders to continuously monitor for arrhythmias over many days, weeks, and months. Woods et al. (2008) used this technique to examine nine subjects trekking to more than 6300 m in Nepal and found that above 5000 m, all subjects showed sinus tachycardia and marked sinus arrhythmia on exercise. One subject had an episode of atrial flutter, one had non-conducted P waves and one had marked ST depression. Of the seven episodes of arrhythmia reported, six occurred during climbing above 4300 m and the seventh was during strenuous exercise at 2800 m when the oxygen saturation was 84%. Prior to the expedition, these subjects did some very strenuous hill walking at low altitude and showed no arrhythmias. More recently the same group used a similar device on 16 British servicemen during an attempt on Dhaulagiri (8167 m) and observed numerous cases of significant sinus pauses and an unspecified form of high-grade heart block (Boos et al. 2017). Additionally, one individual developed atrial fibrillation at 4100 m (282 minutes at a heart rate of 133 bpm) after drinking cold water during the night, and another had a period of supraventricular tachycardia (31 seconds at a heart rate of 207 bpm) on attempting to lift a 30 kg load at 5200 m. While these studies demonstrate that among healthy individuals, the overall rate of arrhythmias is low, it should be noted that the average age of these subjects was only about 30 years and, as a result, the results cannot be generalized to older individuals. In one of the few studies to examine ECG changes in older individuals at high altitude, 97 men and women between 59 and 83 years of age were observed over five days at 2500 m as part of the 10th Mountain Division study. Thirty-nine percent of those studied had ECG abnormalities including Q waves, ST depression, left bundle branch block, and left anterior fascicular block, although many of these changes were present on historical ECGs. Two individuals had new ST segment depression consistent with ischemia, while two manifested new atrial fibrillation (Roach et al. 1995). Sinus arrhythmia accompanying the periodic breathing of sleep is very common at high altitude (Chapter 17). Indeed, the periodic slowing of the heart can be reliably used to identify the presence of periodic breathing at sea level (Guilleminault et al. 1984) and was used in this way with a Holter monitor to detect periodic breathing in climbers at an altitude of 8050 m during AMREE (West 1986). It is likely that the most extreme arterial hypoxemia for a given altitude occurs following periods of apnea during the periodic breathing of sleep. As periods of apnea with hypoxia likely increase both sympathetic and parasympathetic reflex drive to the heart, it is not surprising that occasional premature ventricular and premature atrial contractions are sometimes seen. For example, during four sleep studies at 8050 m, one individual had occasional premature ventricular contractions, another had atrial bigeminy and a third had occasional premature atrial beats (Karliner et al. 1985). To explore these findings, an experimental study using awake voluntary breath holds in humans noted significant bradycardia and arrhythmias including sinus pauses and third degree atrioventricular block after acclimatization to 5050 m (Busch et al. 2018). A prominent role for the arterial chemoreflex was suggested as the underlying cause, as most of these bradyarrhythmias could be relieved with 100% oxygen breathing and the magnitude of bradycardia was related to the individual sensitivity of the hypoxic ventilatory response (Figure 11.6). Figure 11.6Raw data demonstrating apnea-induced arrhythmia at altitude. Examples of electrocardiographic tracings from the same individual during apnea at low (top) and high altitudes (bottom). Apnea at altitude exhibited arrhythmias, such as 3° atrioventricular block (see inset, bottom right). bpm, beats per minute. (Source: Busch et al. 2018.) Acute hypoxia (typically minutes) causes essentially no change in the mean systemic arterial blood pressure in humans, at least up to altitudes of 4600 m (Kontos et al. 1967; Vogel and Harris 1967). This is because despite profound systemic vasodilation and a fall in total peripheral resistance, cardiac output increases to support oxygen delivery in the face of reduced arterial oxygen content (Tamisier et al. 2004). Moreover, during this same time period, sympathetic nervous system activity is elevated (15–200% above baseline, see Figure 11.7), presumably to restrain total peripheral resistance from falling too low (Hansen and Sander 2003; Saito et al. 1991; Sander 2016). Figure 11.7Segments of mean voltage neurograms of muscle sympathetic nerve activity (SNA). Recordings obtained after four weeks at an altitude of 5260 m are in the middle column). Recordings made three days after descent are in the right column and recordings obtained four to six months after return to sea level are in the left column. The neurograms are representative for the three subjects with the lowest (A), median (B), and highest (C) muscle SNA burst frequency at altitude. (Source: Hansen and Sander 2003.) What remains controversial is whether this process represents a perfect balancing act between local vasodilatory signaling mechanisms and increased sympathetic vasoconstriction or blunting of vasoconstrictor pathways, termed hypoxic sympatholysis (Marshall 2015), similar to what occurs during exercise (Remensnyder et al. 1962). During acute hypoxia, the contribution of α-adrenergic vasoconstrictor tone to resting forearm vascular resistance and thus blood flow is greater (Weisbrod et al. 2001), and α-adrenergic responsiveness is preserved. For example, endogenous release of norepinephrine via intra-arterial tyramine infusion caused similar if not greater forearm vasoconstriction in hypoxia (Dinenno et al. 2003). However, these data suggesting preserved sympathetic function are not without controversy. For example, some human studies have observed that hypoxia blunts the forearm vasoconstrictor response to norepinephrine, angiotensin, and lower body negative pressure (Heistad and Wheeler 1970), or have demonstrated a similar change in forearm vascular resistance to lower body negative pressure, but with a greater background of total sympathetic nerve discharge (Rowell et al. 1989; Rowell and Seals 1990). Moreover, hypoxia reduces the magnitude of sympathetically mediated vasoconstriction in the rat through a neuropeptide Y1 receptors mechanism (Coney and Marshall 2007). The discrepancy between studies is not clear, but may be due to both the degree of hypoxia and the choice of pharmacological (local constant drug infusions) or physiologically relevant sympathoexcitatory interventions that alter sympathetic burst patterns (burst frequency and amplitudes). While few mechanistic studies have been performed, it is interesting to note that with advancing age, a situation known for impaired sympathetic vascular transduction in men (Levine et al. 1997), blood pressure actually falls with acute hypoxia (Briant et al. 2016). Finally, syncope occasionally occurs at high altitude in otherwise healthy individuals (Freitas et al. 1996; Nicholas et al. 1992; Perrill 1993; Westendorp et al. 1997). While this has been seen mainly in young adults, often within 24 hours of arrival at altitude, and commonly after a meal including alcohol, an exaggerated increase in circulating epinephrine (Halliwill 2003; Rowell and Seals 1990) alongside blunted sympathetically mediated vasoconstriction is a possible explanation. Indeed, maladjustment of baroreflex function and sympathetic withdrawal seem unlikely, as cardiovagal baroreflex gain is similar between those with and without syncope (Halliwill and Minson 2005). A relatively large number of studies have examined changes in systemic blood pressure in nonhypertensive individuals during more prolonged (hours to months) exposures to high altitude. In the first 10 to 24 hours after arrival at altitude (Sagoo et al. 2017), blood pressure tends to be slightly elevated, but normalizes over the next several days if the altitude is moderate (∼2500 m). At higher altitudes, blood pressure remains elevated despite several weeks of acclimatization (Calbet 2003; Parati et al. 2014; Wolfel et al. 1994). In one study of 32 subjects who moved to an altitude between 3500 m and 4000 m, 31 of them had an increase in resting blood pressure, and this persisted for some three weeks at altitude but returned to normal after descent (Kamat and Banerji 1972). In another study of four sea-level residents who moved to an altitude of 4350 m, the mean arterial blood pressure rose from about 100 mmHg to about 128 mmHg after arrival and this persisted for 10 days (Vogel et al. 1974). One of the noteworthy features of the available data is the large degree of interindividual variability in blood pressure responses. For example, in the study by Palatini et al. (1989), some individuals experienced negligible changes in systolic and diastolic pressure while others saw their systolic and diastolic pressures rise by 17.4 mmHg and 16.3 mmHg, respectively. This variability can also be appreciated in a more recent study by Keyes et al. (2017) in which blood pressure was monitored in normotensive and hypertensive trekkers traveling between 2860 m and 3400 m in the Khumbu Valley of Nepal. Unfortunately, many studies to date suffer from a range of confounding factors that could account for this variability, including the time period after arrival at altitude and the duration of rest prior to the measuring blood pressure, varying restrictions on diet, hydration status and physical activity, and environmental factors (e.g., measurements taken indoors or outdoors). Well-controlled experiments are needed to confirm the physiological relevance of the observed variability and if it exceeds the normal technical and biological variability in blood pressure at sea level. Residing at high altitude for many weeks prompts a complex process of acclimatization (Subudhi et al. 2014). As cardiac output returns to sea level values, the elevation in arterial blood pressure must be due to an increase in total peripheral vascular resistance. Indeed, calf blood flow is reduced and resistance elevated after four weeks at 5260 m (Hansen and Sander 2003). Involvement of sympathetically mediated vasoconstriction is likely, as sympathetic activity remains markedly elevated (∼200–300% above baseline) after four weeks of acclimatization (4100–5260 m) (Hansen and Sander 2003; Lundby et al. 2018; Simpson et al. 2019). Moreover, propranolol attenuates the rise in arterial pressure after 17–19 days at 4300 m (Wolfel et al. 1994), although mean pressure did still increase by ∼8 mmHg, suggesting that other factors are involved. Indeed, while sympathetic activity is high, indirect evidence suggests that the process of acclimatization downregulates the sympathetic transduction pathway, as demonstrated by the fact that systemic phenylephrine infusion causes an attenuated rise in arterial pressure after acclimatization (Simpson et al. 2019). The reason for maintained sympathetic neural overactivity, increased vascular resistance, and higher arterial blood pressure despite successful acclimatization is not entirely known. Acutely, low arterial PO2 stimulates sympathetic activity via peripheral chemoreceptor activation. However, continued peripheral chemoreceptor involvement is not likely, as breathing an FIO2 of 1.0 does not substantially lower sympathetic discharge after four weeks at 5260 m and sympathetic activity remains elevated after return to sea level (Hansen and Sander 2003; Simpson et al. 2019). That being said, one possibility is that hypoxia causes long-term facilitation of either the peripheral chemoreceptor or central brainstem structures that are responsible for setting central sympathetic outflow to a higher set point. Indeed, while the baroreflex retains its capacity to dynamically increase or decrease sympathetic activity after acclimatization to 5050 m, its operating point is higher, allowing for a greater probability of sympathetic firing and therefore a lack of restraint of elevated central sympathetic outflow (Simpson et al. 2019). In contrast to the increases in systemic blood pressure shortly after moving to high altitude, people who reside at altitude for several years apparently have a decrease in both systolic and diastolic pressure (Marticorena et al. 1969). These studies included 100 lowlanders who moved to altitudes of about 3800 to 4300 m for between 2 and 15 years. It has also been demonstrated that a one-year stay at an altitude of 4500 m resulted in a decrease of systemic systolic and diastolic pressures (Canepa et al. 1956), while workers on the Golmud-Lhasa railway had declines in average systolic and diastolic pressures (Wu et al. 2007). Obvious limitations to these findings include the fact that substantial changes in lifestyle and dietary factors may have occurred during the time at high altitude, which could affect blood pressure responses. While much of the attention regarding the cardiovascular system has focused on cardiac function and the microcirculatory aspects of that system, much less work has examined changes in the microcirculation at the tissue level. Martin et al. (2010) examined the sublingual circulation in climbers ascending to Everest base camp and above and found a reduced microcirculatory flow index, a finding also observed during an ascent of Cho Oyu (8201 m) as well as increase in vessel density as compared to sea level (Martin et al. 2009). A follow-up study using a simplified procedure observed increased labial vessel density with short exposure to high altitude, which increased further during prolonged exposure and persisted following descent to Kathmandu (Gilbert-Kawai et al. 2017). The reasons for these observed changes are not clear. A subsequent study using topical nitroglycerin to assess microvascular vascular reactivity established that the observed increase in sublingual vessel density at high altitude is predominantly due to capillary recruitment (Hilty et al. 2019). The overall significance of these changes for resting conditions is not clear, but they could be beneficial in allowing longer transit times and increased oxygen unloading in the tissues. The microcirculation has also been interrogated through estimation of forearm and finger-skin blood flow via laser Doppler fluximetry. Salvi and colleagues (2018) demonstrated an increase in forearm skin microvascular flow motion on ascent to high altitude. Unfortunately, in a similar experiment Davies et al. (2018) found that endothelial and neurogenic forearm flow motion was reduced at high altitude, although finger flow motion was increased. In considering the limited data available thus far about the microcirculation at the tissue level, it is important to note that these studies provide specific insight into the microcirculation of the mouth and skin only. Extrapolation of these data to other vascular beds such as skeletal muscle or other organs should be treated with caution, particularly given how blood flow is often redistributed between organ systems in response to various stimuli such as hypoxia, eating and exercise (Greene and Roach 2004). Given the role of heart-lung interactions in cardiac function and systemic hemodynamics, any discussion of cardiac function at high altitude must also consider changes that occur in the pulmonary circulation. One of the most important physiologic responses to hypoxia is the constriction of pulmonary arterioles in response to alveolar hypoxia, referred to as hypoxic pulmonary vasoconstriction or HPV. This important effect of hypoxia on the pulmonary circulation, which has been reviewed extensively elsewhere (Sylvester et al. 2012), is nicely demonstrated in a study by Barer et al. (1970) (Figure 11.8) in which the left lower lobe of the lung of anesthetized cats was made hypoxic and its blood flow was plotted against the alveolar PO2. The response to hypoxia demonstrated a typical nonlinear stimulus-response curve; when the alveolar PO2 was altered in the region above 100 mmHg, little change in blood flow and vascular resistance was seen whereas when the alveolar PO2 was reduced to approximately 70 mmHg, a marked increase in vascular resistance occurred. Figure 11.8Blood flow from the left lower lobe of open-chest anesthetized cats plotted against the PO2 of the pulmonary venous blood from the lobe. The lobe was ventilated with different inspired gas mixtures while the rest of the lung was breathing air (◦) or 100% oxygen (•). (Source: Barer et al. 1970.) The primary role of HPV is in patients with various forms of lung disease including acute processes such as pneumonia or more chronic diseases like chronic obstructive pulmonary disease or pulmonary fibrosis, whereby vasoconstriction reduces blood flow to poorly ventilated areas of the lung and, as a result, preserves ventilation-perfusion matching, a key to efficient gas exchange. At high altitude, however, the alveolar PO2 is decreased throughout the lung, leading to more extensive hypoxic vasoconstriction. This causes a more uniform topographical distribution of blood flow (Dawson 1972), which is trivial in terms of overall gas exchange (West 1962) and therefore has little physiologic benefit. In fact, depending on the extent to which it occurs, it may actually predispose to complications in select high altitude travelers. In conjunction with the increase in cardiac output seen following ascent, the increase in pulmonary vascular resistance resulting from HPV leads to an increase in pulmonary artery pressure. Comparisons of daily variations in cardiac output and pulmonary artery pressure in normoxia and hypoxia indicate, however, that the bulk of the changes in pulmonary artery pressure are related to the changes in vascular resistance rather than cardiac output (Balanos et al. 2015). There are several noteworthy features about this important physiologic response. The first is that HPV is quite rapid, occurring soon after initiation of a hypoxic exposure (Figure 11.9). Pulmonary artery pressure rises within minutes, followed by further increases over a two-hour period (Frise and Robbins 2015a; Talbot et al. 2005) (Figure 11.10). The second and perhaps more noteworthy feature is the marked interindividual variability in the magnitude of the response at rest and with exercise in hypoxic conditions (Figure 11.11) (Swenson and Bartsch 2012). This has significant clinical implications, as it is those individuals who have an overly large response who are predisposed to development of high altitude pulmonary edema (HAPE, discussed further in Chapter 22) (Dehnert et al. 2005; Grunig et al. 2000). The precise physiological mechanism for this variability remains unclear, but it does not appear to be related to the magnitude of peripheral or central chemoreceptor responses (Fatemian et al. 2016). Figure 11.9Changes in pulmonary vascular resistance (PVR) during control (open circles) and hypoxia protocols (triangles). Open triangles represent values measured during euoxia, whereas closed triangles represent values measured during hypoxia (PETO2 50 Torr). Values are mean + SE. The subscript C refers to the fact that pulmonary vascular resistance was corrected for changes in cardiac output. (Source: Talbot et al. 2005.) Figure 11.10The rise in pulmonary artery pressure (PAP) during eight-hour sustained alveolar hypoxia (PETO2 = 50 Torr) measured using pulmonary artery catheterization in six healthy male volunteers. Squares, systolic PAP; circles, mean PAP; triangles, diastolic PAP. Open symbols indicate measurements in euoxia, while filled symbols indicate measurements in hypoxia. On return to euoxia at eight hours, systolic PAP initially remains elevated; subsequent short hypoxic challenge causes a brisker rise in systolic PAP than that observed at the start of the experiment. (Source: Frise and Robbins 2015a.) Figure 11.11Variability in pulmonary artery pressure (PAP) in high altitude pulmonary edema (HAPE)-susceptible individuals (continuous lines and filled symbols) and in nonsusceptible controls (dashed lines and open symbols) during exposure to normobaric hypoxia (FIO2 0.12). (Source: Swenson and Bartsch 2012.) While studies consistently show that HPV is attenuated by either hypocapnic (Benumof and Wahrenbrock 1977; Brimioulle et al. 1990) or isocapnic alkalosis (Barer et al. 1971; Lyrene et al. 1985), the data regarding the effects of acidosis are mixed with studies showing evidence of enhanced (Emery et al. 1977), unchanged (Brimioulle et al. 1990), or even attenuated HPV (Malik and Kidd 1973). Hyperthermia potentiates HPV (Benumof and Wahrenbrock 1977) while hypothermia attenuates the response (Haavik-Nilsen and Hauge 1968). Data from animal models suggest that HPV may vary by sex. For example, it has been shown that HPV in isolated lungs is greater in male than female sheep (Wetzel and Sylvester 1983) and cats (Cutaia et al. 1987), while studies in isolated rat pulmonary arterial rings treated with phenylephrine demonstrate that HPV is greater in males than females during periods of high estrogen levels. In further support of this concept, Lahm et al. (2008a) have shown that that high doses of exogenous 17β-estradiol rapidly decrease hypoxic pulmonary vasoconstriction in rats in a dose-dependent manner, while Xu et al. (2013) noted less severe hypoxia-induced pulmonary hypertension among those rats exposed to hypobaric hypoxia (PB 380 mmHg) for 21 days and who had higher serum estradiol levels. The precise mechanism for estrogen’s effects is not clear but may relate to upregulation of endothelial nitric oxide synthase, down regulation of endothelin-1 expression and changes in prostacyclin expression (Lahm et al. 2008b). Despite this evidence from animal studies, the issue of sex-based differences in HPV in humans has not been extensively investigated. Docherty et al. (2019) have shown that female human pulmonary artery smooth muscle cells have higher basal HIF-1α expression and lower prolyl hydroxlase-2 levels compared to male cells but the downstream effects on pulmonary vascular responses were not clear from this study. In one of the few other studies to investigate this question, Berendsen et al. (2016) compared isocapnic HPV in seven males and seven females exposed to 20 minutes of hypoxia (PETO2 ∼ 50 mmHg) and found a nonstatistically significant trend toward increased HPV in the female group. This occurred despite the fact that PETCO2 was 5.3 mmHg lower in the females, a surprising result given that hypocapnia typically has a vasodilating effect in the pulmonary vasculature. Further research will be necessary before any firm conclusions can be made regarding sex-based differences in HPV. Several studies have suggested that HPV may be stronger in older individuals. Turner et al. (2015), for example, exposed two groups of individuals (<25 years of age and >60 years of age) to 120 minutes of hypoxia equivalent to 2500 m in a hypobaric chamber and estimated higher systolic pulmonary artery pressures in the older group, although they failed to correct for differences in the alveolar partial pressure of oxygen. More recently, Balanos et al. (2015) exposed 12 younger (mean age 20.5 + 0.5 years) and nine older (mean age 55.8 + 2.1 years) men to 20 minutes of isocapnic hypoxia (PETO2 50 mmHg) and found a greater increase in pulmonary artery pressures in the older subjects (15.2 + 1.3 vs. 9.6 + 0.9 mmHg), which, they attributed to enhanced HPV due to the lack of differences in cardiac output. Differences over longer exposures were not explored in these studies. The essential processes of sensing hypoxia, transducing that signal in the lung, and effecting a response resulting in HPV all occur in the pulmonary artery smooth muscle cell (PASMC). We present here a summary of the processes as they are understood today. More in-depth discussion of this issue is provided in several recent reviews on the topic (Dunham-Snary et al. 2017; Sylvester et al. 2012). Since HPV is initiated locally, and the lung consumes a negligible amount of the oxygen delivered to it via ventilation and blood flow, PO2 stimulates HPV via oxygen tensions in alveolar gas (PAO2) and mixed venous blood
Introduction
Cardiac Function
Cardiac output
Heart Rate
Stroke Volume
Myocardial contractility
Cardiac filling
Increased afterload
Electrical conduction system
Electrical Conduction During Sleep
Systemic Circulation
Systemic Blood Pressure
Changes in Blood Pressure with Acute Exposure
Acute Blood Pressure Regulation
Changes in Blood Pressure with Acclimatization
Blood Pressure Regulation in Acclimatized Individuals
Changes in Blood Pressure with Long-Term Residence
Microcirculation
Pulmonary Circulation
Hypoxic pulmonary vasoconstriction
Physiologic Variables Affecting HPV
Variation in HPV by Sex
Changes in HPV with Aging
Mechanisms of hypoxic pulmonary vasoconstriction
Stay updated, free articles. Join our Telegram channel
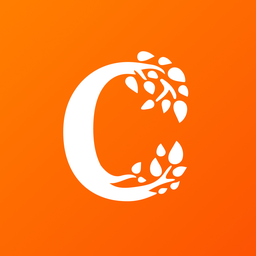
Full access? Get Clinical Tree
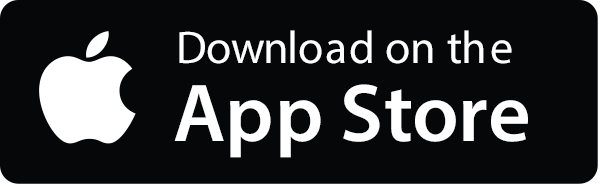
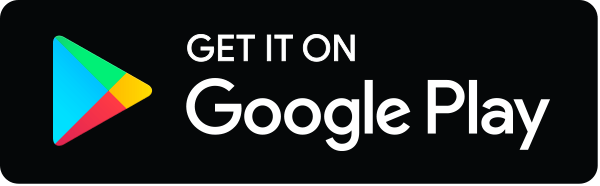